Introduction
Meteorology, an important and interesting branch of Physics in its more copious sense, on account of its extent is more usually treated of separately.
This science has for its object the investigation and explanation of the physical phenomena which occur in the atmosphere, including all those known as meteors, not restricting the term to its more usual acceptation of shooting-stars. Meteoric phenomena may be distributed into various classes, including aerial (winds), watery (water spouts, &c.), optical, fiery, and electrical. The zodiacal light, and many of the shooting-stars which are sometimes embraced under the head of meteorology, in all probability do not belong here, as perhaps not falling within our atmosphere.
A part of meteorology, and one not readily separated from it, is formed by climatology or the theory of climate, by this being meant the geographical part of meteorology, or that which investigates the geographical distribution of those changes and phases of weather, constituting what is called the climate of a place. However great our interest in this subject of the weather and atmospheric phenomena in general, it cannot yet be denied that meteorology is behind all the other departments of physics. This is caused by the great variety and complexity of most of the phenomena, but it is principally because we have not yet been able to collect a sufficient body of laborious, accurate, and long-continued observations from all parts of the earth to make our deductions. Much is due, however, to the labors and researches of A. Von Humboldt, Schouw, Dove, Schübler, Kaemtz, Sabine, Reid, Piddington, Meyen, Redfield, Espy, Hare, and others.
Chemical Constituents of the Atmosphere
The principal components of the atmospheric air are oxygen and nitrogen mixed nearly in the proportion of one to four. To speak more accurately, 100 parts of air contain twenty-one parts oxygen and seventy-nine nitrogen by volume, and twenty-three parts oxygen and seventy-seven parts nitrogen by weight. This ratio is to be considered as constant, and remaining the same in all countries and seasons, without being affected by temperature, moisture, atmospheric pressure, or elevation above the level of the sea. Even in the vicinity of swamps, or in places where large bodies of men have congregated, as in churches, theatres, &c., the proportion of oxygen is the same; whence it follows that the unwholesomeness of such places lies not in the deficiency of oxygen, but in the predominance of some other substance.
Besides oxygen and nitrogen, and the constantly present watery vapor not reckoned as a constituent, there are other ingredients found in the air. some of which are quite variable in quantity. The first of these is carbonic acid gas, produced by the respiration of man and animals, putrefaction, fermentation, combustion, and other processes, and occurring everywhere, at least on land. The amount, according to Humboldt’s observations, is greater in summer than in winter; according to Saussure. greater at midnight than at noon; greater on mountains during dry, windy weather, than in plains when the air is still and damp. Dalton’s observations determined the average amount to be one twentieth, and this was confirmed by Saussure’s experiments made in a meadow near Geneva in 1816–28, who estimated it at 0.049, or nearly one twentieth. The air above the ocean appears to contain but little carbonic acid gas.
Another ingredient of the air is ammonia, a gas generated during the putrefaction of animal matter. This has but recently been detected by Liebig, who found it in snow or rain-water. Rain-water always contains ammonia, and in summer more abundantly than in winter or spring.
The third variable ingredient is hydrogen, hitherto only found as a constituent in the vicinity of volcanoes and swamps, although in the decomposition of animal and vegetable substances, and in the oxydation of metals, considerable quantities must be set free. The supposition of some, that this gas, on account of its extreme lightness, ascends in the atmosphere and forms its confines, is very improbable, as it diffuses itself readily through the pores of other gases. At a height of 20,000 feet Gay Lussac found no appreciable trace of hydrogen.
Distribution of Heat on the Earth

The alternation of heat and cold, and the unequal temperature at different places on the earth’s surface, unquestionably constitute the most important and remarkable changes in the condition of the atmosphere. The sun is to be considered as the principal cause of the heating of the earth’s surface and of the air, his rays producing an effect proportional to the greatness of the angle of their incidence, although this effect may be modified by the density of the strata and the absorbing power of terrestrial objects. It is necessary, however, to consider the double motion of the sun (the diurnal and the annual) in its meteorological relations. In the diurnal motion of the sun, he stands highest in all places at noon, and at this time, or a few hours later, the heat is generally the greatest. Nevertheless, the height of the noon-day sun is neither the same at the same place throughout the year, nor the same at all places on the same day. It is only between the tropics that the sun at noon stands vertically over head, and this only once in the year at each tropic, and twice at the equator. The deviation from perpendicularity during the rest of the year is, however, so slight, that this region (between 23° 28′ N., and 23° 28′ S. lat.) is naturally the warmest on the earth, deserving the name of torrid zone. In those countries of the earth lying about the poles and within the polar circles, the sun is above the horizon for a day, or even for days, weeks, or months (six months at the poles), during a certain portion of the year, and below it for equal lengths of time during another portion, the length of these uninterrupted days or nights increasing towards either pole. Nevertheless, as the sun in these regions can never ascend far above the horizon, his rays must always fall very obliquely in the two frigid zones. The rest of the earth’s surface lying between the tropics or polar circles, or extending from 23° 28′ to 66° 32′ north and south latitude, forms the two temperate zones, north and south. These include more than one half, or .52 of the entire surface of the earth; the two frigid zones about a twelfth, or over .08; and the torrid zone almost .40.
The two temperate zones have four seasons (spring, summer, autumn, and winter), these commencing at different times in the two zones. For the north temperate zone spring commences March 21, when day and night are everywhere equal; summer on June 21, when the days are longest; autumn on September 23, when day and night are again equal; and winter on December 21. when the days are shortest. The seasons of the south temperate zone are precisely the reverse of these just mentioned, summer and spring of one answering to winter and autumn of the other. From March 21st to September 23d, the sun is north of the equator, and traverses from west to east the six signs, Aries, Taurus, Gemini, Cancer, Leo, Virgo; in the rest of the year he passes through the remaining signs, Libra, Scorpio, Sagittarius, Capricornus, Aquarius, and Pisces. He is in the equator March 21st and September 23d, at which time places on the equator have him in the zenith. (See pl. 23, fig. 1, where the dotted circle indicates the equator; the circle intersecting it in two points, and marked with the signs of the ecliptic, the ecliptic; and the irregular curved line, the temperature in the course of the year. This figure is known as Howard’s diagram.)
As a general rule, the further from the equator the greater is the difference between the summer and winter temperature; even in the vicinity of the polar circles the summer may be very hot. This depends upon the influence exerted by the unequal length of days. This difference is very slight at the tropics, where the inequality of days is inconsiderable. Thus at the equator the days and nights are equal throughout; at a latitude of 8
- 14 hours at 30° 48′
- 15 hours at 41° 24′
- 16 hours at 49° 3′
- 17 hours at 54° 31′
- 18 hours at 58° 27′
- 19 hours at 61° 19′
- 20 hours at 63° 23′
- 21 hours at 64° 50′
- 22 hours at 65° 48′
- 23 hours at 66° 21′
- 24 hours at 66° 32′.
The longest day, with a slight allowance for refraction, is equal to the longest night of a place, and the shortest days and nights are found by subtracting the hours of the above table from twenty-four. The astronomical determination of the seasons is not quite appropriate for meteorology, this rather requiring spring for the northern hemisphere to begin March 1st; summer June 1st; autumn September 1st; and winter December 1st. The case is just the reverse in the southern hemisphere, mid- winter there corresponding to mid-summer here.
In the torrid zone the year is divided into two seasons, the dry and the wet or rainy. The latter commences when the sun at noon approaches the zenith, and therefore at different times of year on the opposite sides of the equator. The previously clear sky becomes clouded, and rainy weather sets in, which continues with little interruption for several months. In the interior of Africa each of these seasons lasts just six months. In the frigid zone the year is likewise divided into two seasons, winter and summer, which yet are of very unequal duration, the former having the preponderance in proportion to the proximity of the pole.
Nevertheless, the heat experienced at a given place, at a given time of the year or day, does not depend on the geographical position of the place or the situation of the sun alone, but also on many other circumstances. It is therefore of great interest to ascertain the variation of temperature of a place by continued and frequent thermometrical observations. If the thermometer, which for this purpose must be placed in the open air, and protected from the direct rays of the sun, and from radiation, reflection, &c, be examined hourly, it will be found that the minimum of daily temperature generally takes place fifteen or twenty minutes before sun-rise, the maximum some hours after noon, and later in summer than in winter; in summer between two and three o’clock, in winter between twelve and one. The mean temperature of the twenty-four hourly observations is very nearly that of the highest and lowest temperature, or that of several corresponding hours in the morning and evening, as four and ten in the morning and evening. The temperature between nine and ten A. M., that at sunset, and that at eight P.M., is also very near the mean.
Self-registering or maxima and minima thermometers are used to ascertain the highest and lowest temperatures in a given time, without the necessity of constantly observing the instruments. The self-register invented by Rutherford consisted of two thermometers in the same frame, with their tubes horizontal, one of these being filled with mercury, the other with colored alcohol. In the tube of the former is a cylindrical steel pin, which is pushed forwards by the expansion of the mercury, but does not return with its contraction, thus registering the highest temperature. In the tube of the alcohol thermometer is a fine glass rod, somewhat thicker at the extremities, which retains its place when the liquor expands, but is retracted with the latter on its contraction by a diminution of the temperature. The bulbs of the two thermometers lie in opposite directions on the stand. To adjust the apparatus for a fresh experiment it is to be gently inclined, the alcohol bulb uppermost, and slightly tapped. The steel pin slides down to the top of the mercury, and the glass rod to the end of the column of spirit. These indexes are now so placed that an increase of temperature causes the steel to advance, its diminution producing the retraction of the glass. This instrument is not well calculated for travellers, being principally adapted to fixed stations, and is quite inapplicable to ascertaining the maxima and minima of temperature in mines, caves, Artesian wells, depths at sea, &c. The description of such an instrument, invented by Magnus, and called the geothermometer, will be found under the head of Mining.
The mean temperature of the month is obtained by taking the mean of all the mean daily temperatures; and that of the year will be the mean of the mean monthly temperatures. The maximum of cold occurs about the 14th of January; that of heat about the 26th of July. The mean annual temperature is experienced about April 24th and October 21st. At Paris, during the interval from 1665 to 1823, the coldest day fell principally in the second half of January. At Frankfort on the Maine, the average of twenty years’ observations gave January 15th as the coldest and July 22d as the warmest days, the days of mean annual temperature occurring about April 8th and October 18th. In the torrid zone there are two maxima and two minima in each year. The former occur at the equator about April 20th and October 20th; the latter about January 20th and July 20th. The observed maxima and minima differ in Surinam by 52°, in Pondicherry by 73°, and in Cairo by 88°F. This difference, again, is at Rome 111°, at Paris 142°, at Prague 145
The mean temperature of a place is obtained by taking the mean of as many annual means as possible; this may, however, be approximately obtained from observations of a few years, the mean annual temperatures of a place differing little from each other. Thus, the mean temperature of Moscow and St. Petersburgh amounts to 37
The first clear view of the distribution of heat over the surface of the earth was given by Humboldt, by means of his isothermal lines, lines connecting all those places whose mean annual temperature is the same. These are shown in pl. 29, fig. 1. as laid down on Mercator’s projection, with the thermometrical degrees by fives of Centigrade; this projection also exhibits the proportion of rain. That isothermal obtained by connecting the hottest places of the earth, whose mean temperature is about 82
Nevertheless it is not sufficient to know the mean annual temperature of a place to be acquainted with the distribution of heat in the different seasons, since places on the same isothermal line do not necessarily present the same climatic relations. Thus, Edinburgh and Tubingen have the same annual temperature of 47
The climate of a country, besides its geographical position, depends upon many circumstances, as the proximity of the sea and of mountain ranges; the peculiarities of the soil, &c., exercise a great influence. We distinguish a land and a sea (island) climate; the land is warmed and cooled more readily and more quickly than the sea. For this reason the daily and annual variations of temperature are much more considerable in the interior of continents than over the sea, on the coast, and over islands and peninsulas, which have a more constant climate. Tobolsk and Irkutsk, in the interior of Asia, have summers like those of Berlin; to these, however, follow severe winters, in which the mean temperature of the coldest month amounts to from −
The temperature of the ground is often remarkably different from that of the air, sometimes higher and sometimes lower, according to circumstances. The mean temperature of the earth’s surface agrees very nearly with that of the air at a mean latitude, and is generally indicated by the temperature of springs; at higher latitudes the mean temperature of the ground is higher, and at lower latitudes it is lower than that of the air. The temperature of the ground from the equator to the pole diminishes the faster as we approach the parallel of 45°. At a slight depth the variations of temperature are much less than at the surface, and at still greater depths there is no variation whatever, a constant temperature existing, but little different from the mean annual temperature of the place. The depth at which all annual variations of temperature vanish entirely, varies in different places; in the torrid zone it amounts to only one foot; in the temperate, as in France, Germany, &c., this depth is from sixty to seventy feet; even here, however, the diurnal variations vanish at a depth of from one and a half to three feet. The temperature of the earth increases regularly with increasing depth, this increase on an average amounting to about one degree for every forty or fifty feet, although the exact law of this increase has not yet been determined. Its cause is to be found in the original heat of the earth, which, radiated from the surface, is still retained in the centre. Should the heat increase in the same ratio with increasing distance from the surface, water would boil at a depth of 10,000 feet, and granite would melt at a depth of five miles; consequently, the heat at the centre of the earth must be so great as to melt even the most refractory bodies. The reason that this heat is not sensible at the earth’s surface is to be found in the badly-conducting character of her crust.
The opposite phenomenon, a decrease of heat, is observed as we ascend in the air, the higher layers being colder than the lower: nevertheless, the diminution of temperature is not exactly proportional to the elevation. As the lower strata of air become heated by contact with the earth, they ascend, and expanding as they ascend, a great amount of heat is rendered latent; the temperature is thus necessarily reduced. On high mountains, particularly those of Central and South America, this decrease in temperature is well shown by the change in the vegetation as we ascend. Thus on the slope of a single mountain we may pass in comparatively few hours from the climate and vegetation of the tropics to the stunted flora and icy temperature of polar regions. The precise diminution of temperature depends upon the character of the particular mountain on which it is observed. The variation of temperature experienced in ascending in a balloon differs from that on high mountains, the latter absorbing heat during the day, which is again radiated or transmitted to the incumbent layers, thus elevating the temperature. Elevation and other circumstances being equal, it is warmer on elevated planes and connected mountain ranges than on isolated mountains; on the former the periodical variations of temperature are also greater. Gay Lussac, in his celebrated balloon ascension, found that the temperature diminished on an average about one degree Fahrenheit for every 615 English feet of ascent. In the Cordilleras the elevation necessary for a decrease of one degree F. in temperature was about 300 feet (French) from to 3000 feet of elevation; 444 feet from 3000 to 9000; 228 feet from 9000 to 12,000; and about 306 feet from 12,000 to 15,000: the average of all was thus about 318 feet to the degree. From a comparison of the temperature of Geneva and the St. Bernard, an elevation of 335 feet was found to correspond on the average to a decrease of one degree of temperature; nevertheless, this ratio varies in different months very considerably. In other regions of the Alps the ratio is less than that just mentioned.
At a certain height above the level of the sea, varying with the latitude, a temperature prevails so low in degree, that ice and snow once formed do not melt, but remain throughout the year. This snow line, or the greatest, height up to which snow can melt, is higher as we approach nearer the equator. The following table of snow lines is taken from Humboldt; the elevations are in English feet.
Mountain Chains | Latitude | Elevation |
---|---|---|
Cordilleras of Quito | 0°–1 |
15,730 |
Cordilleras of Bolivia | 16°–17 |
17,060 |
Cordilleras of Mexico | 19 N. | 15,030 |
Himalaya Northern side | 31 | 16,940 |
Himalaya Southern side | 31 | 12,470 |
Pyrenees | 43 | 8,950 |
Caucasus | 43 | 10,870 |
Swiss Alps | 46 | 8,760 |
Carpathians | 49 | 8,500 |
Altai | 50 | 6,395 |
Norway Interior | 70 | 3,512 |
Norway Coast | 71 |
2,302 |
In illustration of the subject let the reader refer to pl. 23, fig. 2, where NESQ is the earth’s surface. CDEF the outer boundary of the atmosphere, ANBS a section of the limit of perpetual snow, which, apart from single irregularities, attains its greatest height, AE and BQ, in the equatorial regions, coming down to the surface of the earth near the poles. It is a great error to suppose that the snow line always lies in those regions where the mean annual temperature is 32° F.; it almost always lies higher than this. Its altitude depends principally upon the temperature of the hottest month, and also upon the local moisture, the shape of the mountains, &c., and is far from increasing regularly towards the equator. Hence it not rarely occurs that the snow line is higher on one mountain than on another which lies nearer the equator. Thus in Norway this line lies proportion ably very high, being 400 feet higher at a latitude of 70° than in the islands 5° further south. As a general rule the snow line lies lower on the coast of a country than in its interior. Under the equator the snow line of South America reaches the height of Mont Blanc; fourteen to eighteen degrees south of the equator in the Chilian Andes, according to Pentland, it rises 2500 feet higher than under the equator, as at Quito, Chimborazo, Cotopaxi, and Antisana. The reason of the lesser height of the snow line on the north side of the Himalaya than on the south (see preceding table) is to be found in the deposit of immense quantities of rain and snow on the south side, from the atmosphere charged with the vapors of the Indian Ocean, far less falling from the dry air of the northern declivity. Even in the Alps there is a great difference in this respect between the south and north sides. Saussure observed that the great fields of snow in the Alps were capable of depressing the snow line by as much as 600 feet. In some cases these curves in the snow line are very numerous, as shown in pl. 23, fig. 3.
While we have a means of examining the lower snow line, owing to its accessibility, we have none for the upper limit, because the mountains of the earth do not lift themselves into those regions where, on account of the too great rarity and dryness of the air, snow no longer presents itself. The term upper snow line, as used by some authors, is not to be understood in the sense just referred to. It simply means the limit of perpetual snow: while the lower snow line refers to a limit at which snow sometimes continues for some years.
But little change in the temperature of springs is observed with the different seasons, the range in the temperate zones being rarely more than from 1°–3°F. Their highest temperature in the northern hemisphere occurs in September, their lowest in March. In the torrid zone their mean temperature is somewhat lower, in the temperate somewhat higher than that of the air. Springs coming from great depths have, however, a much higher temperature, as shown by various salt and mineral springs, and artesian wells.
As already observed, the daily variations of temperature are much slighter at sea than on land. In the torrid zone, the difference between the daily maximum and minimum, at the highest, amounts to 2°–4°F.; in the temperate zone to 3°–6°F. In the torrid zone, the temperature of the sea decreases with the depth; while at the surface this may amount to from 80°–88°F., at great depths it may be only 35° or 36°F. This coldness of the lower strata of the ocean waters, according to Humboldt, causes currents which lead the polar waters towards the equator.
Atmospheric Pressure
The amount of pressure exerted by the atmosphere at any one place is measured by the height of the mercury in the barometer. Temperature, however, also affects this height, the mercury rising slightly with increase of heat, and sinking with cold, independently of any change in the pressure of the air. To compare different observations, therefore, it becomes necessary to reduce them to some standard of temperature, this being assumed at 32°F., or the freezing point. Now mercury expands .0001 of its bulk for every degree above 32°F., therefore all that is necessary to make the required reduction is to subtract the ten -thousandth part of the observed altitude for every degree of Fahrenheit above 32°. Application may also be made of the formula h − (t − 32) × h + .0001 = the corrected height, where h = the observed height of the mercurial column, and t = the temperature at the time of observation. Allowance must also be made for the expansion of the attached scale, unless this be of glass, brass, or other metals expanding nearly as much as mercury. A third correction must be made for capillary depression of the mercury. For the sake of avoiding tedious calculations, tables of these corrections required have been constructed.
The barometer exhibits incessant variations in altitude, distinguished into diurnal and annual. Of these the former are much less conspicuous in the temperate zones than in the torrid. There the amplitude or greatest extent of daily variation is, in Quito, 2.82, in Guinea 2.44, in Cumana over 2
On taking the mean of the hourly variations of the barometer for every month, it will be found that in June, July, and August, the barometer is generally higher in the morning and lower in the afternoon than the mean annual temperature, while the opposite is the case in October, November, and December. The mean monthly barometer height varies from one month to another, and this more conspicuously in the temperate than in the torrid zone; it is higher in winter than in the other seasons. The accidental, or not periodical variations, are considerably greater in winter than in summer, and this the more with the distance from the equator. Lines connecting places of equal annual variation of the barometer are called isobarometrical lines. These are not parallel to circles of latitude, but ascend, for instance, northwards from the eastern coast of America towards Europe and Asia, diverge in the interior of the continent of the old world more and more from the equator, and then sink down again.
The absolute mean barometric condition of a place, like the mean temperature, is obtained by taking the mean of as many mean annual barometric heights as possible. This depends not only on the level above the sea, but also on the geographical position in longitude and latitude. For this reason the mean barometer height is not, as formerly supposed, the same everywhere at the level of the sea. It increases from the equator in either direction, attaining its maximum between 30° and 40° of latitude, and then decreasing to between 60° and 70°. It appears again to ascend within the polar circle. From the equator to 15° of latitude, the mean height of the barometer at the level of the sea amounts to 337–338 Paris lines (29.65 to 29.74 English inches), it then increases to 339 lines (29.83 inches), afterwards decreasing. The mean height of the barometer at the level of the sea also depends to a certain extent on the longitude, at equal longitudes and seasons being greater in the Atlantic than in the Pacific, the difference being on the northern hemisphere 1.3 lines in winter and 1.8 lines in summer, and in the southern 0.3 lines in summer and 1.6 lines in winter. The following table exhibits the mean height of the barometer at different places situated near the sea:—
- Equator 0° 336.5‴
- Christiansborg 5
° N 336.9‴ - Cumana 11° N 336.9‴
- Callao 12° S 337.2‴
- Madras 13° N 337.3‴
- Peru 17° S 337.35‴
- St. Thomas 18° N 337.1‴
- Rio Janeiro 23° S 338.7‴
- Macao 22° N 338.2‴
- Madeira 32
° N 339.1‴ - Cape of Good Hope 34° S 338.2‴
- Naples 41° N 338.2‴
- Marseilles 43° N 337.4‴
- Triest 46° N 337.8‴
- Brest 48° N 338.5‴
- Paris 49° N 337.5‴
- London 51
° N 337.2‴ - Edinburgh 56° N 336.1‴
- South Sea 57
° S 336.4‴ - Stockholm 59° N 335.9‴
- St. Petersburg 60° N 337.2‴
- Reikiavig 64° N 333.4‴
- Gothaab 64° N 331.5‴
- Spitzbergen 75–78&° N 335.5‴
Erman assumes the reduced mean barometer height at the equator at 337.2″; at a latitude of 25° he calculated the maximum to be 338.7; at a latitude of 45°, to be 337.6‴. (The line, indicated by (‴), is .088 of an English inch.)
The cause of the above-mentioned variations of the barometer is to be found in the unequal distribution of heat on the earth, this varying incessantly. Heated air being lighter than that which is cooler, causes a difference in atmospheric pressure, as indicated by the barometer. Suppose the air over a certain region to become heated: the column expands, and rising higher than the surrounding atmosphere, is diffused laterally. Under this column, then, the mercury will fall, rising under the colder surrounding atmosphere, over which the excess of the ascending column had spread.
From what has just been said it is clear that the falling of the barometer, at least in Europe, is generally connected with a rise of the thermometer, and vice versa. This same connexion between the rise and fall of the two instruments takes place in the tropics, for which reason the barometer may be called a differential thermometer, from its indicating the difference of temperature between neighboring great tracts of country. The barometer may, however, rise and fall considerably without any change in the thermometer, and both instruments may rise or fall together. The regular changes of temperature in the course of the day have little influence on the barometer. The maximum of the irregular variations of the barometer occurs when the yearly temperature is the least; the minimum a short time before the occurrence of the highest temperature.
The barometer, it is well known, is sometimes used to predict the weather. As a general rule, the barometer by day sinks before a rain, and ascends during the rain. It exhibits more or less agitation during storms, and from this we may draw conclusions with respect to such storms, and even sometimes predict them; yet the proposition that the barometer must stand very low during storms is not always true. During the storm of Dec. 14, 1786, on the Isle of France, the barometer fell nearly eight lines in four hours, and ascended again ten and a half lines in two hours. During the hurricane on Sept. 21st, 1819, at St. Thomas, it fell four lines in four hours, and again ascended more than five lines in three quarters of an hour. From Humboldt’s observations the hurricanes between the tropics are not accompanied by so great a depression of the barometer as is generally supposed.
Of the Winds
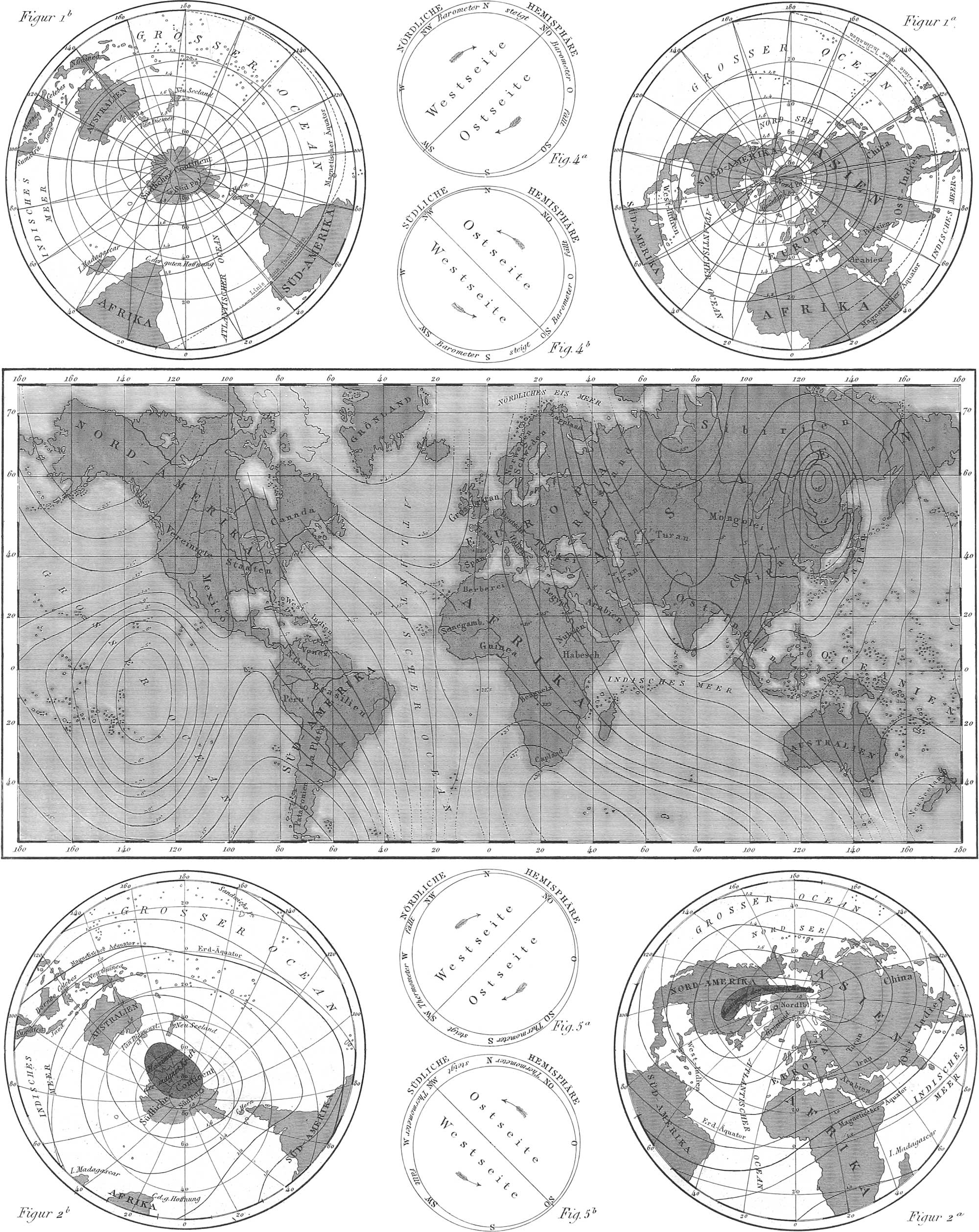
Glossary for plate 28
- Ægypten, Egypt.
- Æquator-Grenze des Schnecfalls, Equatorial boundary of the snow region.
- Aleuten, Aleutian Islands.
- Arabien, Arabia.
- Asien, Asia.
- Barometer steigt,—fällt, Barometer rises,—falls.
- Berberei, Barbary.
- Bestdädiger Eegen, Perpetual rain.
- C. der guten Hoffnung, Cape of Good Hope.
- Canarische Inseln, Canary Islands.
- Capverdische Inseln, Cape Verd Islands.
- Deutschland, Germany.
- Erd-Æquator, Terrestrial equator.
- Europa, Europe.
- Frankreich, France.
- Freundschafts Inseln, Friendly Islands.
- Gebiet der Monsun Regen, Region of the Monsoons.
- Gesellschafls Inseln, Society Islands.
- Grönland, Greenland.
- Grossbritanien, Great Britain.
- Grosser Ocean, Pacific Ocean.
- Herbst und Winter Regen, Autumn and winter rains.
- Indisches Meer, Indian Sea.
- Italien, Italy.
- Kopenhagen, Copenhagen.
- Kurve von Leith, Br., Curve of Leith, latitude.
- Kurve von Padua, Br., Curve of Padua, latitude.
- Linie ohne Inclination, Line without inclination.
- Magnetischer Æquator, Magnetic equator.
- Manschurei. Mandshoo territory.
- Maximum der magnet. Kraft, Maximum of magnetic power.
- Mittlere Tageswärme, Medium daily temperature.
- Mongolei, Mongolia.
- N. O., N. E.
- Neufundland, Newfoundland.
- Neu Guinea, New Guinea.
- Neu Sealand, New Zealand.
- Niedrige Inseln, Low Islands.
- Nord Amerika, North America.
- Nördlicher Gürtel der beständigen Niederschläge, Northern zone of perpetual deposits.
- Nördliche Hemisphäre, Northern Hemisphere
- Nördlicher Polarkreis, Arctic Circle.
- Nördliches Eismeer, Arctic Sea.
- Nordpol, North pole.
- Nord See, North sea.
- Norwegen, Norway.
- Nubien, Nubia.
- Oceanien, Oceania.
- Ost Indien, The East Indies.
- Ostseite, East side.
- Persien, Persia.
- Provinz des Herbstregens;—des Sommerregens;—des Winterregens, Region of autumnal, of summer, and of winter rains.
- Regenloses Gebiet, Rainless region.
- Russland, Russia.
- Schwacher Sommerregen, Light summer rain.
- Sibirien, Siberia.
- S. O., S. E.
- S. O. Monsun im Apr.–Oct., N. W. Monsun im Oct.–Apr., S. E. Monsoon from April to October, N. W. Monsoon from October to April.
- Spanien, Spain.
- Sud Amerika, South America.
- Südpol, South pole.
- Südlicher Continent, Southern continent.
- Südlicher Gürtel der beständigen Niederschläge, Southern zone of perpetual deposits.
- Südliche Hemisphäre, Southern Hemisphere.
- S. W. Monsun im Apr.–Oct., N. O. Monsun im Oct.–Apr., S. W. Monsoon from April to October, N. E. Monsoon from October to April.
- Thermometer steigt,—fällt, Thermometer rises,—falls.
- Turkei, Turkey.
- Vereinigte Staaten, United States.
- Wärme-Æquator, Equator of heat.
- Wendekreis des Krebses;—des Steinbocks, Tropic of Cancer;—of Capricorn.
- West Indien, The West Indies.
- Westseite, West side.
- Winterregen, Winter rains.
- Wüste Schamo oder Gobi, Desert of Shamo, or Gobi.
- Zone häufiger, fast beständiger Niederschläge, stets mit electrischen Explosionen, Zone of frequent, nearly perpetual deposits, always accompanied by electrical explosions.
All motions of the atmosphere, or aerial currents, are known by the general term winds. These almost always arise from an unequal heating of the earth’s surface and of the incumbent air, although other causes may occasionally operate, as, for instance, the sudden condensation of watery vapor in the atmosphere. The theory of winds is well illustrated by an experiment as figured in pl. 23, fig. 62. If, during winter, the door of a warm room leading into an apartment or passage-way that is not heated, be slightly opened, and a burning candle held to the upper end of the crevice, the flame will be driven outwards, and thereby demonstrate the existence of a current of heated air from the upper part of the room. About the middle of the opening the flame will be vertical, owing to the absence of both an inward and outward current. At the bottom, again, a current will be again sensible, consisting of the colder external air pressing into the room to supply the loss occasioned by the upper outward current. This air then, after entering the room, becoming heated, and therefore lighter, ascends and passes out at the top again. Precisely in the same manner the air in the warmer regions of the earth ascends and flows over the colder, while that from the surrounding colder regions flows in from below.
To determine the direction of winds at the surface of the earth, weather-cocks or wind-vanes are used. These consist of a flat thin piece of sheet iron or other material, of an appropriate shape. This is placed in a vertical plane, and turning on a vertical axis passing through its centre of gravity. The surface of the vane on one side of this axis must be greater than on the other, so that when struck by the wind it may have a determinate direction. Thus we may construct the vane in the shape of an arrow broadly feathered; the barb will then point in the direction from which the wind comes. This direction is generally estimated by the eye, but may be obtained more accurately by fixing under the vane a series of rods, connected in a framework, and pointing to the four quarters of the horizon. Each rod has a letter at its extremity indicating this direction as N., S., E., W.; sometimes there are four others to indicate the N.W., S.W., N.E., S.E. points of the compass. A very convenient construction of the vane is to have its axis passing down through the roof into a chamber. On the lower end of the axis is to be fixed an index, which shall rotate in a circle marked with the directions of the wind. This index, turning simultaneously and equally with the vane, will always tell the direction of the wind without the necessity of going outside of the house. It should have been before mentioned, that vanes, to be of scientific value, must be as much as possible free from the minor currents produced by local obstructions; their elevation, then, above surrounding objects, is absolutely necessary. Self-registering weathercocks, called anemometers, or anemographs, have also been constructed by Landriani, Parrot, Traill, Osier, and others, where the direction of the wind during the twenty-four hours is traced directly on paper without the necessity of an attendant.
Besides the direction of the wind it is necessary to consider its velocity and strength. The simplest method of ascertaining the velocity of wind is to make use of an apparatus similar to that employed in ascertaining the velocity of a current of water. Thus, a light body, as a piece of paper or a downy feather, &c., may be let loose into the air, and the distance traversed in a given time noted; this is, however, entirely inapplicable in high winds, owing to the irregularity of the motion, and the difficulty of properly regulating the experiment. Some have endeavored to use the passage of the shadows of clouds over the surface of the earth to ascertain this velocity, but this method is not very applicable, owing to the uncertainty of the path and the small portion measurable by a single observer. The most satisfactory and generally used method is to ascertain the perpendicular pressure of the current against an opposed surface of known area, assuming that the force of the wind is a consequence or a function of its velocity. Contrivances for measuring the force of the wind are called anemometers, in the restricted sense of the term. They have been proposed and constructed in great variety. The first method proposed was to allow the wind to strike against a vertically depending disk or plate, and to determine its force by the angle through which the plate was lifted. This idea was the basis of the anemometer of Pickering and Oertel. In Dalberg’s construction, the disk, instead of depending, was erected vertically on a hinge, and kept to the wind by a great vane. More recently, Parrot has proposed instead of a disk to take a hollow ball, which, suspended freely by a rod, shall be raised by the wind, the angle of elevation being measured on an attached quadrant, and from this angle the force of the wind determined. One of the oldest and simplest anemometers is that of Bouguer, consisting of a square plate fastened to a four-sided rod. This rod fitted in a hollow four-cornered parallelopipedon, and pressed against a spiral spring contained in it. The plate being made to face the wind, the amount of pressure exerted on the spring was measured by an index. This instrument has recently been much improved by Beaufoy.
In another class of anemometers the wind turns wheels or vanes: to these belong those of Christiani, Wolf, Leutmann, and others, the ingenious self registering anemometer of Michael Lomonosow, and the hydrometric vane of Waltmann. By means of this latter instrument the velocity of running water may be ascertained as well as that of the wind. It consists of a small windmill, to whose axis an endless screw is attached, which catches in the teeth of a wheel; the axis of the latter is provided with an index for the purpose of measuring the entire number of revolutions of the mill. Among the more recent instruments the portable one of Lind (probably invented by Hales) deserves special attention, on account of the small surface presented to the wind, large surfaces always involving great uncertainty. This consists of two parallel glass tubes about eight or nine inches long, half an inch in diameter, and connected beneath by a bent tube of one tenth of an inch bore. A metal cap, bent at right angles, is attached to the upper end of one tube, its opening receiving the wind. Both legs are so fastened to a vertical iron rod as that the wind keeps the whole instrument in the direction of its current. Water is poured into the tubes, filling them about half full. When the wind blows into the mouth of the metal cap, it depresses the water in one tube, causing it to rise in the other. The difference of level between the two tubes gives the height of a column of water whose weight is equal to the force of the wind acting on a surface of equal area. This anemometer gives approximately very accurate results. There are, however, three difficulties in the way of its general use: unequal temperature, evaporation, and the freezing of the water; on this latter account it were better to make use of dilute sulphuric acid.
According to the determinations of Rouse, the velocity of an almost insensible wind amounts to one and a half feet in a second; of one just perceptible, three to four and a half feet; of a pleasant wind, six to seven and a half; of a brisk wind, fourteen and a half to twenty-two; of a very brisk wind, twenty-nine and a half to thirty-six and a half; of a strong wind, forty-four to fifty-one and a half; of a very strong wind, fifty-eight and a half to sixty-six; of a storm, seventy-three and a half; of a violent storm, eighty-eight; of a hurricane, 117; and of the most violent hurricane, to 147 feet per second. Brandes estimated the velocity of a moderate wind at twelve to sixteen feet; Woltmann, that of a violent storm at seventy to eighty feet; Rochan, that of a tropical storm at 150 feet; the maximum being 600 feet. Kraft determined the velocity of the wind during a violent storm in St. Petersburg to be 110 to 123 feet. Finally, the wind appears to increase in velocity with the elevation.
Kaemtz has divided the winds into regular and irregular or variable. To the first class belong the land and sea breezes, the trade winds, and the monsoons. Land and sea breezes are experienced on coasts and islands, and are produced by causes already explained. After sunrise the land is heated to a greater degree than the sea; consequently, about ten A.M. a current sets in from the sea—a sea breeze—which reaches its maximum of intensity between two and three P.M., a calm again ensuing about sunset. Equilibrium of temperature between the two is soon disturbed by the greater depression of temperature on land produced by more rapid radiation; this being now cooler than the sea, a current of colder air pours out seawards from the land—the land breeze—which lasts until about eight A.M. In both cases an opposite current exists in the higher regions of the atmosphere. The land breeze is inconsiderable about peninsulas, but very sensible in bays. Similar periodical variations prevail also on the banks of great lakes, as also in long valleys and ravines.
It very frequently happens that currents of air, at different heights, move in entirely different directions, as shown by the motion of clouds of different degrees of elevation. This is illustrated by pl. 24, fig. 12, where ab is the direction of the lower, dc that of the upper current. In investigations into these currents, small balloons, like those used so successfully by Thomas Forster, are very useful; in most cases four to five, and sometimes even seven to eight simultaneous different currents may be found to exist. According to the observations of Placidus Heinrich, instituted at Regensburg (Ratisbon) in the months of May, June, and July, 1791, the
- North wind blew 9 times above, 11 times below
- N.E. wind blew 11 times above, 12 times below
- E. wind blew 7 times above, 10 times below
- S.E. wind blew 3 times above, 9 times below
- S. wind blew 5 times above, 5 times below
- S.W. wind blew 15 times above, 14 times below
- W. wind blew 76 times above, 40 times below
- N.W. wind blew 19 times above, 44 times below
The trade wind, which in the torrid zone blows constantly from east to west, may be readily understood from what has already been stated. In the vicinity of the equator, the air being greatly heated, expands and flows above towards the poles, at the same time that a counter current of cold air sets in from the poles to the equator. Had the earth no rotation on an axis, there would be a north wind on the northern hemisphere, and a south on the southern. But the earth turns on her axis from west to east once in twenty-four hours, and individual regions have an actual velocity which is greater with the vicinity to the equator. The air coming from the poles, and preserving its original velocity, must move slower towards the east than the earth itself, and consequently will have, in respect to the latter, a relative motion from east to west. This motion, combined with the motion from the poles to the equator, gives as its resultant a north-east wind to countries north of the equator, and a south-east wind to those south of it. These winds blow over the whole earth, but are generally only sensible at sea, at some distance (about fifty miles) from the main land. In the Atlantic the trade wind extends from 8° to 28° or 30°; in the Pacific to 25° N.L.; the limits in the southern hemisphere are less accurately determined. Sailors make use of these winds in the voyage from Europe to America, by steering south from Madeira nearly to the tropic of Cancer, whence, with little effort of the crew, they are carried west. In like manner the Spanish vessels went regularly from Acapulco to Manilla with this wind. Kaemtz assumes the limits of the north-east trade wind at 2° and 23° N. (the latter moves in summer a little more northerly, and in winter a little more southerly), that of the south-east trade at 2° to 4° and 21° S., the northern limit of the latter lying in the Atlantic Ocean in the northern hemisphere. In the vicinity of the equator the two trades become more easterly, and are separated by a zone in which there is generally an almost total absence of wind, hence called the region of calms. This has a mean breadth of about 6° (in August of 9
The upper current from the equator to the poles has, in the northern hemisphere, a south-westerly, in the southern, a north-westerly direction. The altitude at which this current commences has not yet been determined; on Teneriffe it prevails at an elevation of 9000 feet, while Humboldt found the easterly trade wind on South America at an altitude of 8000 feet. In all cases, the equatorial current becoming cooler, sinks deeper and deeper, finally reaching the surface of the earth. Thus in the higher latitudes the two opposite currents meet, and mutually interfere without any regular alternation taking place between them. On the great seas the west winds are tolerably regular between 30° and 40°, more so in the southern than in the northern hemisphere, and are used in the voyage to the Cape of Good Hope. In northern and western Europe the south-west winds decidedly predominate. The same is the case on the Atlantic, between Europe and North America, for which reason the voyage from Europe to America generally lasts longer than that from America to Europe. According to the recent calculations of Mahlmann at the mean latitude of the temperate zone, in both continents, a west-south- w r est wind is to be considered as the prevailing one See the chart of the winds (pl. 47. fig. 1) illustrating what has just been said.
The following table gives the number of times, that is, the number of days in the 1000, during which, on an average, each one of the eight principal winds blows in the countries mentioned in the table:
Countries | N. | N. E. | E. | S. E. | S. | S. W. | W. | N. W. |
---|---|---|---|---|---|---|---|---|
England | 82 | 111 | 99 | 81 | 111 | 225 | 171 | 120 |
France | 126 | 140 | 84 | 76 | 117 | 192 | 155 | 110 |
Germany | 84 | 98 | 119 | 87 | 97 | 185 | 198 | 131 |
Denmark | 65 | 98 | 100 | 129 | 92 | 198 | 161 | 156 |
Sweden | 102 | 104 | 80 | 110 | 128 | 210 | 159 | 106 |
Russia | 99 | 191 | 81 | 130 | 98 | 143 | 166 | 192 |
N. America | 96 | 116 | 49 | 108 | 123 | 197 | 101 | 210 |
The season of the year has a great influence upon the direction of the wind. In Europe the direction is more southerly during winter than in the other seasons; in spring (March and April) east winds occur, in summer (particularly in July) west and also north winds, and in autumn (especially in October) south winds. The strength of the wind depends partly on the season, being greatest in winter (January and February), and partly on the time of day, increasing in the morning towards noon, and then decreasing towards evening.
In Europe the wind when changing appears to move for any one place from north by east, south and west, as seems to have been first suggested by Lord Bacon, and after him by Mariotte, Sturm, Lampadius, Schubler, and others. The same relation seems to exist in North America. Dove first attempted to investigate this succession, and attained to the following results: In that part of the northern hemisphere where equatorial and polar currents alternate, the wind, as a general rule, shifts from the south by west, north, and east, and returns between south and west, as also between north and east, more frequently than in other directions. In the southern hemisphere the wind changes from south by east, north, and west, coming back more frequently between north and west, south and east. In the temperate, and probably in the frigid zones, where equatorial and polar currents constantly alternate, the wind at a mean appears to go round the quarters of the horizon in a definite direction, this direction being opposite in the two hemispheres. In the torrid zone, where polar currents alone prevail at the surface of the earth, there is no complete rotation of the wind. Nevertheless, whenever in the torrid zone, owing to the particular distribution of land and sea, a northern current alternates once a year with a southern, there will be but one complete rotation in the course of the year, and that in the direction which predominates in the south temperate zone.
The direction of the wind, as already remarked, exerts a decided influence on the height of the barometer. This, in western and central Europe, is highest during northern and eastern, lowest during southern and western winds. The following table contains the height of the barometer corresponding to the various winds, from observations made in the places mentioned:
London Millimetres | Paris Millimetres | Berlin Millimetres | Carlsruh Paris lines | Dantzig Paris lines | St. Petersburg Eng. inches | Moscow Millimetres | |
---|---|---|---|---|---|---|---|
N. | 759.2 | 759.1 | 758.7 | 334.7 | 338.7 | 28.06 | 743.1 |
N.E. | 760.7 | 759.5 | 759.4 | 335.0 | 338.6 | 28.15 | 745.1 |
E. | 758.9 | 757.2 | 758.8 | 334.5 | 338.8 | 28.15 | 743.9 |
S.E. | 756.8 | 754.0 | 754.7 | 333.5 | 338.8 | 28.16 | 741.7 |
S. | 754.4 | 753.1 | 751.3 | 332.8 | 337.7 | 28.07 | 740.6 |
S.W. | 755.2 | 753.5 | 752.6 | 333.4 | 336.3 | 28.07 | 740.3 |
W. | 757.3 | 755.6 | 756.0 | 333.7 | 337.1 | 28.05 | 741.1 |
N.W. | 758.0 | 757.8 | 756.6 | 334.3 | 337.9 | 28.00 | 741.5 |
Curves have been constructed for different places, showing the dependence of the barometer on the winds, as shown in pl. 28, figs. 4a and 4b. Now as the height of the barometer is in a certain connexion with the temperature, the latter must stand in a certain relation to the direction of the wind. In Europe, the temperature of the air is generally lower during northern than southern winds, and lowest of all during north-east winds; this rule, however, exhibits many occasional exceptions in different places and seasons. Thus, in Paris, during summer, it is coldest during south-west, west, and north-west winds, and warmest during south-east winds; in winter, on the other hand, it is coldest during north, north-east, and north winds, and warmest during south, south-west, and west winds. Curves exhibiting the connexion between the condition of the thermometer and the directions of the wind for a given place, are called by Dove thermometric wind rosettes (figs. 5a and 5b).
Unusually high winds or storms are caused by some great disturbance of the equilibrium of the atmosphere, which, in many cases, is produced by a rapid condensation of watery vapor, and the consequent rarefaction of the air; the air then rushes violently from all directions to the place of rarefaction, the place itself changing its position. This theory is maintained by Brandes and Espy. According to Redfield and Dove, storms are to be considered as whirlwinds, having a north-eastward progressive motion from the south to the north of the tropic of Cancer, the air in the whirl rotating simultaneously in a given direction. In the northern hemisphere this is from south to north by east. In pl. 23, fig. 63, AB represents the line along which the minimum of atmospheric pressure progresses. The circles described about A and B represent the whirl at the beginning and at the end of the storm. At the commencement of the storm, south-east or south winds will prevail at places south-east of AB; south-east at D and E; south at C and F; at the end of the storm the wind will have a westerly direction at these places. To the north-west of AB the wind will blow in the opposite direction. In the southern hemisphere the whirlwinds move from the north-west, and have the opposite direction of rotation.
Coasts and islands are especially subject to violent storms, owing to the absence of the mountains and elevated ranges which so eminently alleviate the force of the wind on the main land. On the coast of the North sea, the storms occur principally in November, and are preceded by calms and by an uncommonly damp warm atmosphere. Violent storms also occur on the coast of Norway, coming principally from the north-west, also ushered in by uncommonly warm weather. On the coast of Finmark, thirty-three storms were counted in the single year 1798; only twenty-six occurred, however, at another time during a period of twelve years. In the north frigid zone storms from the south-west and south are not rare; the most violent come from the south, shifting round to the north. Among the fiercest storms are found those which the mistral (north-east wind) produces quite frequently on land, on the Mediterranean, and even as far south as Africa. One of these, occurring on the 13th of October, 1782, pressed against an opposing surface with a force of 12lbs. to the square foot.
The most terrible storms of all, called tornados, trovados, or hurricanes, occur in the torrid zone, particularly in the West Indies, where they produce at times appalling devastation. They take place nearly every year, beginning generally in the tropical regions, passing, however, out of these, and generally sparing the islands of Trinidad and Tobago, which are protected by high mountains; this, however, is not always the case, as has been generally believed. A peculiarity attending them is that the wind, during the continuance of the storm, changes its direction, generally blowing from all parts of the compass in succession; quite frequently, also, during the storm there occurs a lull, followed by a change of the wind to the opposite direction. Among the most devastating hurricanes of this century belongs that of 1809 in the channel of Mozambique, and on the island of Bourbon, Mauritius, and Rodrigues, as also that of August, 1837, which devastated the coasts of the main land of North America and the Lesser Antilles. Pl. 47, figs. 9 and 10, represent their sphere of influence. The explanation of these figures will be found in another part of the work.
Still more severe was the tornado of October 10th, 1780, which destroyed the fleet of Lord Rodney and a great number of merchantmen, killed 9,000 men in Martinique and 6,000 in St. Lucia, and levelled to the ground the towns of St. Pierre in Martinique, and Kingston in St. Vincent (fourteen only out of six hundred houses being left standing in the latter place). There was also a storm at Guadaloupe, in July 25, 1825, in which the wind appeared to be luminous; and the one of August, 1831, which, beginning on the 16th at Barbadoes, extended within six days over the islands of St. Vincent, St. Lucia, Granada, Martinique, Porto Rico, Cuba, and as far as New Orleans, destroying at Barbadoes alone ten millions of dollars’ worth of property, besides the lives of 2500 men. Many tornadoes extend into the United States, particularly the southern portion of it, generally, however, losing a great part of their violence. Furthermore, the rainy season in the torrid zone, in Africa particularly, is connected with storms which often pass into veritable hurricanes. On the west coast of Africa, especially on the coast of Sierra Leone, two or three tornadoes form the transition from the wet to the dry season; these are most severe when unaccompanied by rain, and are then called white tornadoes. Very violent storms also rage on the east coast of South Africa, to which the Mauritius is exposed; the most devastating were in the years 1760, 1761, 1766, 1772, 1773, 1786, 1789, 1818 (when forty ships were torn loose from their anchors and mostly shattered to pieces, and many men on the island were destroyed), and 1824. The Cape of Good Hope is a dangerous neighborhood, on account of its storms, the most fearful of which are those which strike Cape Town. The storms on New Holland are also very severe, and during their continuance the wind blows from all quarters of the horizon.
The whirlwinds occurring in the seas of China and Japan, and devastating these coasts, are called typhoons. Resembling the hurricanes of the west Indies in their rotary motion, and the calm succeeded by the opposite wind, they differ in having a less conspicuous rectilineal progression.
The hot winds form a peculiar class, embracing the samum, the harmattan, the chamsin, the sirocco, and the solano. The three first appear to be identical; samum, also simum, semum, simoom, or samiel (poisonous wind), is the name of this wind in Arabia, Persia, and in most countries of the east. It is called chamsin in Egypt, and harmattan in the negro tongue. The term chamsin, meaning fifty, comes from its blowing generally for the fifty days from April 27 to June 18, although sometimes the vernal equinox occurs in the middle of its period. These winds show themselves in greatest violence in treeless, sandy deserts, their distinguishing character being heat and dryness. A darkening of the horizon is the surest precursor of the simoom; when it actually arrives, the clear heaven vanishes, the sun loses his lustre, the air is filled with particles of sand, and all nature exhibits signs of the greatest disquiet. The heat becomes much more oppressive during the storm, particularly when columns of sand are carried along by the current. That the wind in itself is deadly, or at least injurious to the health, seems to be unfounded; at any rate the narrations of many of the earlier travellers appear to have been highly overcharged. Even the assertion that men and camels cast themselves on the ground at the approach of the storm, burying their faces in the sand, and thus await the passage of the blast over them, appears to belong to the class of fables; so much is true that the Arabians, during these storms, not unfrequently veil their faces and kneel down behind their camels. In western Asia, particularly in Arabia Petrsea, and the deserts between Bagdad, Bassora, Aleppo, and Mecca, the simoom blows only during the months of June, July, and August, being most violent in July; it presents itself only by day, and on land, lasting at most only a few hours. With regard to the direction of these winds, they come on the borders of the desert from the following quarters: in lower Egypt from the south-west, in Mecca from the east, in Surat from the north, in Bassora from the north-west, in Bagdad from the west, in Syria from the south-east, &c. On the western coast of Africa, particularly in Senegambia, the wind is called harmattan; it there is injurious only to vegetation, being directly conducive to health by driving away fevers and other diseases. The chamsin in Egypt generally lasts from two to three days.
The sirocco of Italy, and the solano of southern Spain, are probably only continuations of the harmattan blowing in Africa, which loses its dryness in passing over Mount Atlas and the Mediterranean sea, retaining, however, its heat and relaxing properties. The influence of the sirocco, principally exerted in Sicily and Naples, and the isle of Malta, sometimes extends to southern Germany (the Tyrol and Steyermark). On the island of Madeira it blows as an east-south-east wind; it is there remarkably dry, accompanied by cloudless skies, and lasting about three days. The solano in Spain is likewise remarkable for its heat.
The Föhn which comes down from the Alps into some of the valleys of Switzerland is also supposed to be a continuation of the sirocco. It is a south wind, exceeding all other winds of the country in violence, before whose occurrence the sun becomes dimmed, and a haze forms over the sky. Shortly before the beginning of the föhn a north wind called föhn-bise generally blows in the higher regions. When the föhn approaches, plants wither, animals become restless, and the inhabitants experience a sensation of weakness and relaxation. In spring it furthers the progress of vegetation in a remarkable degree, and causes the snow to melt very rapidly. It is most violent on the Lake of the Four Cantons, where it sometimes tears up nets lying deep in the water. It lasts generally but for a few hours, occasionally, however, for eight or more days; it blows with greatest intensity in spring and autumn, rarely in winter, and very seldom in summer. This wind was very remarkable in its appearance on the 18th of July, 3 841, when it extended at the same time over a great part of Germany, even reaching as far as the Black Forest and the Odenwald.
The Mistral of South France cannot be considered as a wind allied to the sirocco and föhn; it is rather a very violent north-west wind, generally dry and cold, extending as a north wind as far as Algiers.
The hot winds in the south-eastern part of New Holland are very remarkable; these come from the high mountains in the north and north-east, and hardly yield to the hottest winds of Africa in their temperature.
Cold winds are less conspicuous and frequent than warm. They are felt in long valleys, and come from northern side valleys, and are especially cold when these contain ice and snow the year round. Winds of this character are principally found in Thibet, and are called Burana when they are stormy. In the deserts of Beloochistan they are accompanied during the summer months by copious falls of rain. The cold storms of the Russian Steppes, called Wiuga, are exceedingly devastating, being accompanied by snow squalls, and lasting generally three days.
Whirlwinds arise from the meeting of two winds blowing from opposite directions; these sometimes, even on calm days, set sand, dust, &c, into a whirling motion, frequently lifting these objects to a considerable height. Very violent whirlwinds produce the so-called trombs or weather columns, also called wind or water spouts, as they occur on land or over the water. The name tromb (French) is borrowed from the trumpet-shape of the object. In every phenomenon of this nature there is a kind of cloud which descends in a hollow column from the clouds above to the earth, or to the surface of water. This progresses with varying velocity, rotating on a vertical axis, and taking up water as well as dry substances, which it sometimes elevates to the height of several hundred feet. These spouts generally form a hollow, straight, or curved double cone, whose upper part, with the point downwards, proceeds from a cloud; the lower part, with the point directed upwards, consisting of water, sand, and other substances. The color is generally grey, dark blue, dark brown, and sometimes fiery red; the middle portion is frequently transparent. The force exercised by these spouts is, in many cases, truly enormous; they uproot trees, unroof houses, hurl men and other objects to a great distance, &c. The motion of the spout is often very rapid and tempestuous, some traversing seven or eight miles in an hour, while others can readily be followed on foot; they rarely, however, stand still. Horner asserts the following propositions with regard to water-spouts proper. They arise for the most part only in the vicinity of land where inconstant winds blow and the temperature is very variable, and are always accompanied by thunder and lightning, or electrical phenomena. Around them calms generally prevail. They carry along with them all objects taken up. They arise sometimes from the clouds, sometimes from the water; their mass consists of watery vapor, and not of solid water; their greatest diameter varies from 2 to 200, their greatest height from 30 to 3000 feet. A figure of a water-spout is seen in pl. 27, fig. 1. Land whirls, according to Horner, have the same origin with water-spouts; they are, however, more impetuous and destructive, not being retarded or impeded by the weight of water. Peltier has found recorded 116 whirls, of which sixty belonged to the land, and fifty-six to the sea. Of the former the oldest was in 1456, of the latter in 1664. Of the entire number twenty-nine (eighteen by land and nineteen by sea) exhibited a circular motion, and twenty-two (thirteen by land and nine by sea) no internal motion at all; forty-one (twenty-five by land and sixteen by sea) were accompanied by thunder and lightning; ten carried the objects taken up in a direction opposite to the wind; sixteen ended with hail; six vanished in a cloudless atmosphere without doing any damage. Of the water-spouts, three shook fresh water over the ship, although on the sea; in fifteen the water was seen to rise; in eight to sink down; two connected an upper cloud with a lower; in eight cases a sulphureous smell was perceived; in six cases several spouts were seen at the same time; thirty-four exhibited peculiar phenomena.
Of the Moisture of the Atmosphere
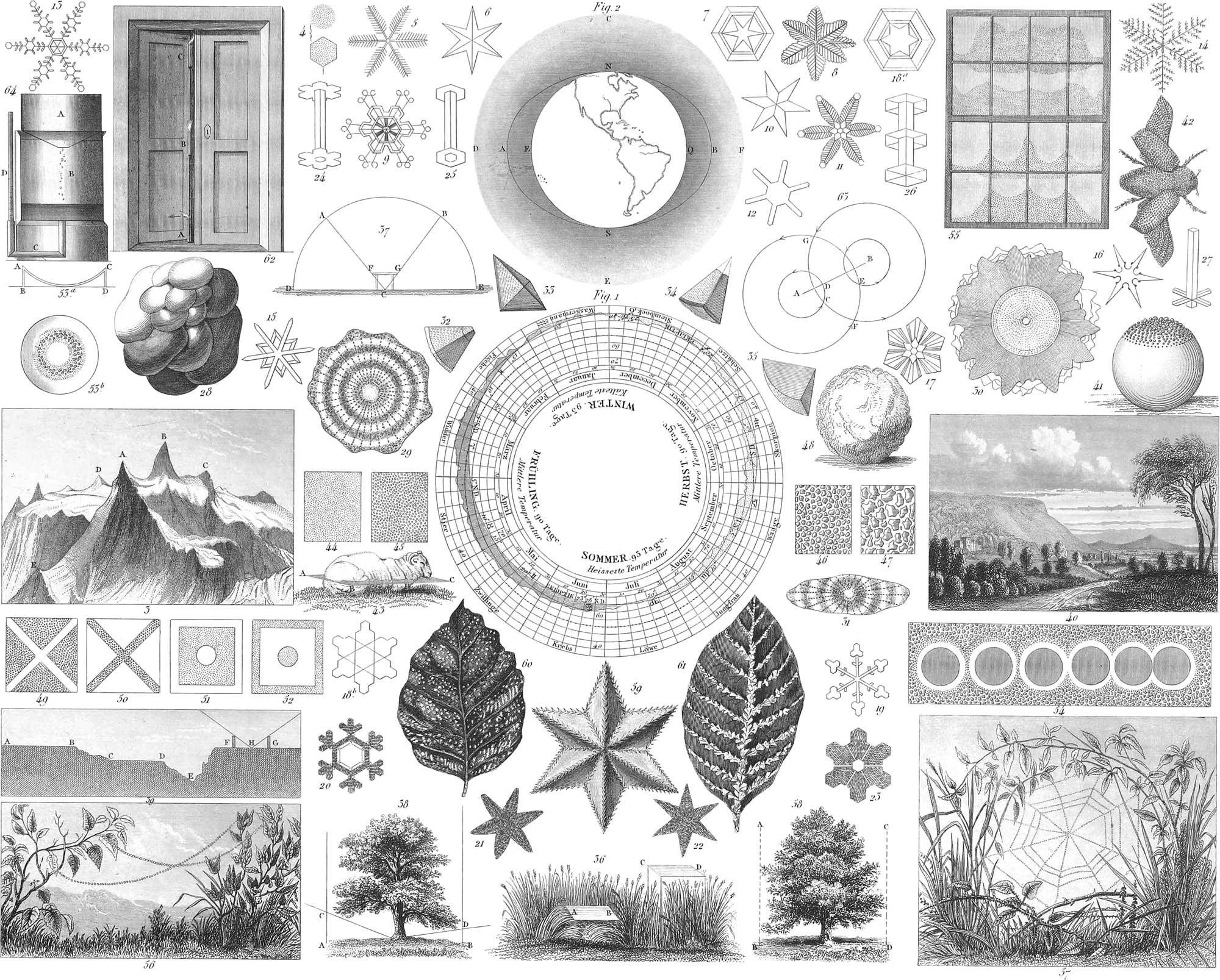
Glossary for plate 23
- Februar, February.
- Fische, Pisces.
- Frühling 90 Tage, Spring 90 days.
- Heisseste Temperatur, Hottest temperature.
- Herbsi 90 Tage, Autumn 90 days.
- Januar, January.
- Juli, July.
- Jungfrau, Virgo.
- Juni, June.
- Kälteste Temperatur, Coldest temperature.
- Krebs, Cancer.
- Lowe, Leo.
- Mai, May.
- Marz, March.
- Mittlere Temperatur, Medium temperature.
- Schütze, Sagittarius.
- Skorpion, Scorpio.
- Sommer 93 Tage, Summer 93 days.
- Steinbock, Capricornus.
- Stier, Taurus.
- Waage, Libra.
- Wassermann, Aquarius.
- Widder, Aries.
- Winter 93 Tage, Winter 93 days.
- Zwillinge, Gemini.
A greater or less quantity of water is always dissolved in the air in the form of vapor. This vapor, under ordinary circumstances, is invisible, but becomes visible when it returns to its liquid state in the form of dew or frost, fog or cloud, rain or snow. Certain instruments, called hygrometers, are employed to determine the amount of watery vapor contained in the air. The hygrometers formerly employed had for their basis some organic or inorganic body, which became elongated or increased in weight by absorbing watery vapor, the amount of absorption being in proportion to the amount of vapor in the atmosphere. The best instrument of this kind was the hair hygrometer of Saussure. A soft pale human hair is boiled for half an hour in a solution of sulphate of soda, and then for a few minutes in pure water, washed off in cold water, and dried in the shade. It is then fastened by its upper extremity to a little tongue, the other end being wound round a minute pulley provided with two grooves. Round the other groove passes a silk thread, from which is suspended a weight of several grains to keep the hair in a state of constant tension. An index, fastened to the pulley, traverses a graduated arc whenever the pulley is turned in either direction by the contraction or expansion of the hair. To graduate the instrument and fit it for its proper office, it is first introduced into a receiver, in the bottom of which has been placed heated chloride of calcium or concentrated sulphuric acid, and after the air has been exhausted, the place noted where the index stands; this will be the point of greatest dryness, or the of the scale. It is next placed in a receiver provided with a basin of distilled water, and the new position of the index marked: this will indicate the maximum of moisture, or the 100th degree of the scale. The interval between the two extremes is then to be divided into one hundred equal parts. Other substances employed in hygrometers are from the animal kingdom: 1. Catgut, as used in the Dutch hygrometers, consisting of a paper house in which is suspended a piece of gut. The lower end of the gut carries a disk of paper, on which are fixed two figures, one a man with an umbrella, the other a woman with a fan; the former comes to the door when the moisture causes the string to turn, and the latter when this is untwisted by the dry air. 2. Quills, as proposed by Chiminello. 3. The skin of the frog, fish bladders, gold beaters’ skin, silk, ivory (employed by Delue, who afterwards substituted whalebone), &c., &c. The vegetable kingdom has furnished hempen threads, thin boards of pine or mahogany, or box wood, paper (especially straw paper), grasses, awns of oats, and a species of erodium. From the mineral kingdom have been derived solutions of sal-ammoniac, in which a sponge is dipped, salt, sulphuric acid, &c., &c. All these, however, are inapplicable to the purposes of science, owing to their great variability and the readiness with which they lose their hygrometric properties.
The hygrometer of Daniell is constructed on an entirely different principle from the preceding, namely, condensation and rarefaction. This consists of a curved exhausted glass tube, ending in two bulbs, the one having a thin coating of gold or platinum foil, the other covered with a fine linen rag. The first bulb is half filled with sulphuric ether, and contains a small thermometer, the graduated portion of which passes up into the tube. On dropping ether on the other bulb, its rapid evaporation produces a considerable degree of cold; the vapor of ether in the tube becomes condensed, thereby permitting a new evolution from the ether in the bulb. The formation of vapor in this bulb cools it by rendering some of its heat latent, thus causing it to become covered with a delicate dew. The dew point can be read off on the inclosed thermometer, that is, the temperature at which condensation or the deposit of vapor begins, this taking place when the air is saturated with moisture. The reason that the dew point can serve to determine the amount of watery vapor in the atmosphere is found in the following considerations: The air is said to be saturated with moisture when the vapor contained in it has attained the maximum of tension and density, corresponding to the existing temperature, which is not always the case, for the warmer the air, the greater may be the tension and density of the vapor contained in it. When moist air is cooled, it is incapable of containing as much vapor as before; a portion then becomes condensed in the form of minute vesicles. This condensation will take place sooner with a given reduction of temperature, the more vapor is contained in the air, or the nearer it is to the maximum of condensation corresponding to the existing temperature. Upon the same principle depends a more perfect instrument, invented by Dobereiner, and recently improved by Regnault.
All hygrometers depending on the principle just mentioned, are subject to the defect of causing a great expense of ether, and of not allowing any condensation at all when the weather is very dry. August’s psychrometer is far preferable, and in all probability will soon supersede other hygrometric apparatus. The principle is the same as that proposed by Hutton, and employed by Leslie in his differential thermometer. The psychrometer consists of two very sensitive thermometers, attached or freely suspended to the same frame; of the two bulbs, one is wrapped with a linen rag, the other is free. On moistening the first bulb, without leaving any water dripping, an evaporation takes place, which is rapid in proportion as the air is removed from the point of saturation. This evaporation reduces the temperature of the inclosed bulb, and the mercury sinks in proportion as the air is removed from the point of saturation. Thus from the difference of temperature in the two thermometers we can draw a conclusion as to the condition of moisture in the atmosphere. The psychrometer thus does not give the dew point itself, but the point of greatest density, that is, the temperature at which the external air is so saturated with vapor, as to be incapable of taking up an additional amount without condensation occurring with a further reduction. By the use of certain formulae we can avail ourselves of the indications of the psychrometer in determining the dew point itself.
The amount of moisture in the air varies through the day. According to the researches of Kaemtz and others, it twice reaches a maximum and twice a minimum; the former occurs about 9 A.M. and 9 P.M.; the latter shortly before sunset, and about 4 A.M. Nevertheless, in a certain sense we may say that the air is most damp about sunrise, as it is then coldest, and nearer the point of saturation or the dew point than at any other time of day. We do not then mean that the air contains absolutely the most moisture at this time, but most in proportion to the temperature. In summer the minimum of moisture is at about 3 P.M. On high mountains the changes in the condition of moisture in the air follow another law, the ascending currents carrying the vapor upwards. This condition also varies with the month and season. From observations instituted in London, Paris, Geneva, and the great St. Bernard, the absolute amount of vapor at those places was least in January, and greatest in the end of July or beginning of August; on the other hand, the relative moisture was greatest at the three first named places in December, and least in May (beginning of May at Geneva, end of May at London and Paris). The summer months are then, with respect to the relative moisture, the dryest, and the winter months the dampest. The elasticity of the vapor is likewise different at different seasons for the same place, being subject to many variations, and particularly to the influence of the wind. Dove has shown from DanielPs observations, that the pressure of the vapor atmosphere is much less during north and south winds than during eastern and western; Kaemtz ascertained, likewise, from observations made at Paris in 1827, that on an average the minimum of pressure lay in north-east winds, the maximum in those a little west of south; in summer, however, considerably east of south. The relative amount of moisture in the air also varies with different winds; on an average the air is further removed from the condition of saturation during north-east winds, than during southern and south-west winds, although occasionally decided exceptions to these general principles occur.
As the formation of vapor depends partly upon the temperature and partly upon the presence of water, it follows that the dryness of the air must increase with the distance from the equator and from the sea, an inference well established by observation.
A partial condensation of the vapor of the air takes place whenever it is brought into contact with a sufficiently cold body, whose temperature is lower than that which corresponds to the density of the existing vapor. A part of the latter is thus deposited on the cold body in the form of minute vesicles. The moister the air the less the temperature of the body needs to differ from that of the air. In this way we explain the coatings of moisture on the window panes of an inhabited room, whose temperature is higher than that of the external air, so that the panes are coated from within, a phenomenon which is only exceptionally reversed, and this especially in spring. Pl. 23, fig. 55, shows the symmetrical manner in which these vesicles are deposited.
Of Dew
The local deposit of moisture referred to well illustrates the theory of dew in general. When after sunset the sky is clear and the air calm, a great radiation of heat takes place from the earth, accompanied of course by a diminution of temperature. The layers of air in contact with the earth and objects upon it become cooled, and when this reduction of temperature reaches the dew point, a deposit of vapor ensues.
To determine the amount of dew we make use of a drosometer, an instrument still very imperfect and inadequate. By drosometer is to be understood a balance, the shorter arm of which carries a plate, readily bedewed, the other arm a counter weight. Instead of the plate, as used by Wilson and Flaugergues, we may use to advantage a bunch of wool or eider-down, to be appended to the shorter arm. Wells, to whom we are indebted for the first rational theory of dew, used flocks of wool weighing ten grains, pulling them into a mass of about two inches diameter, and measuring their increase in weight after exposure. Lambert proposed to expose well washed and curly hair to the air, and then to ascertain its increase of weight in a given time.
The following generalities respecting dew are furnished by Wells. Dew is formed in greater quantity during clear calm nights, especially when clear and calm weather succeeds to stormy. Clouds prevent the formation of dew, as also brisk winds, which constantly replace the cooled air by warmer. Little dew falls during a night when the sky is cloudy and the wind still; none at all when a cloudy sky and windy weather are conjoined. Objects interposed between the radiating surface and the clear sky. produce the same effect as clouds; consequently, bodies standing freely exposed to the heavens, exhibit the greatest deposit of dew. Even a piece of paper, or a fine pocket handkerchief, will prevent the deposit of dew. A bundle of wool lying underneath a piece of paper gained only two grains of dew, while another of equal size, but exposed freely to the sky, gained sixteen grains, or eight times as much. Fig. 36, pl. 23, presents an apparent exception to the law. Lay a metal plate, AB, on high grass, heavy enough to press down the grass and thereby to become surrounded by it, and at the same time support a similar plate, CD, on thin props above the average height of the grass, thus exposing the plate to the open sky. The first metal plate will in many cases be bedewed, while the second is found to be entirely free from moisture. This result is unquestionably produced by the cooling influence of the grass on the former plate. The action of an oblique exposure to the sky is shown by another experiment (pl. 23, fig. 37). Under the elevated board, FG, lay a small quantity of wool at C, ten grains for instance; on account of the board this cannot radiate heat towards the part AB of the sky, it will therefore be less bedewed than if it had been freely exposed. Around the stem and under the branches of a tree less dew falls than in the surrounding free exposure. In the case represented by fig. 38, little dew will be deposited in the space represented by AB, determined by verticals tangent to the outer circumference of the branches. Differently inclined surfaces experience different deposits of dew. Let fig. 39 be a vertical section of a certain region of considerable unevenness, while apart from this the radiating power is everywhere the same; then more dew will be deposited on the horizontal portion, AB, than on the slope, BC; more also than on CD. The least deposit will be at E. If a body be placed at H, on the same level with AB, it will be less covered with dew, on account of the intercepting action of the walls F and G, than if it lay on AB. Two observers would obtain very different results, one of whom should be stationed on a well wooded and inclosed slope, like the foreground of fig. 40, and the other on the open height in the back-ground of the same picture.
An instructive experiment is performed by suspending a glass ball at some height above the ground. In a perfectly calm clear night the first moisture will be exhibited on the very summit of the ball; by degrees the dew drops will extend over a′ great portion of the ball, constantly diminishing in size, as in pl. 23, fig. 41. A similar action is observed on a bent sheet of paper, a dead insect (fig. 42), and on the back of a sheep lying quietly on the earth (fig. 43); in the latter figure the greatest horizontal section, ABC, indicates the boundary between the more and the less bedewed portion.
Since different bodies possess very different radiating properties, and consequently cool in a very unequal degree, it follows that under precisely the same circumstances the deposit of dew on these different bodies would be very different. Thus on plants it is more copious than on the earth; on grass and leaves more than on bushes and trees; on loose gravelly land more than on the hard-trodden soil; on glass more than on metals, &c. Plane and horizontal pieces of glass are well adapted for observing the formation of dew. Pl. 23, figs. 44–47, show how the drops, small at first, afterwards increase and run together. Wool deserves the preference so far, that besides readily receiving the dew, it retains it for some time. The amount of dew produced depends not so much on the quantity of wool as upon the manner of its arrangement. A certain quantity of wool rolled up in a ball (fig. 48) will receive much less dew than if spread out flat. Metals become dewed very slowly, although there is a difference in this respect, platinum, iron, steel, and zinc, receiving a greater amount than gold, silver, copper, and tin. Metals purposely moistened sometimes become dry while other bodies are becoming coated. If a metal be combined with a substance of some thickness, capable of being strongly bedewed, the radiating power of the metal is not increased, as might be supposed, but diminished, and the resistance to the deposition of dew presented by the metal may even be communicated to other bodies lying upon it. Thus wool on a plate of metal becomes less wetted than if lying on a plate of glass. If, however, a piece of gilt paper be laid with its unmetallic side upon wood, the paper will be perfectly dry where it is in contact with the wood, and the metallic side will become bedewed (figs. 49–52).
If a watch-glass be laid on a plate of polished tin, with the concave side up (pl. 23, figs. 53a and 53b), a dry zone will be seen on the outer border of the glass, and a circular dry space in the centre, the two separated by a zone of dew, exhibiting the largest drop in the middle. An example of the regularity with which dew is deposited around a row of wafers laid on glass, themselves receiving no dew, is seen in fig. 54. Finally, figs. 56 and 57 show the dewing of spiders’ webs.
According to the experiments of Wells, it is not correct to assign morning and evening as the time of greatest deposit of dew. It seems rather to be deposited during all hours of the night, more abundantly, perhaps, after midnight than before it. In shaded places it appears to form even in the afternoon. Nevertheless dew is not deposited in all countries in equal quantities. It is most copious in the coast regions of warm lands; for instance, along the Red Sea, the Persian Gulf, the coast of Coromandel, at Alexandria, and in Chili. On the other hand it is almost entirely wanting on the arid plains in the interior of continents, as in central Brazil, in the deserts of Sahara and Nubia, &c. It is very rarely seen at sea.
That dew does not ascend, as supposed by the earlier philosophers, is now not a matter of controversy. Other things being equal, the amount of dew will be in proportion to that of moisture in the atmosphere, for which reason heavy dews are frequently the precursors of rain. With equal quantities of moisture in the air the dews of cold nights are more copious than those of warm. Dew-water, when collected, is found to be almost chemically pure, containing, however, some carbonic acid. The sticky deposits found on plants, called honey-dew and meal-dew, do not proceed from the atmosphere, but consist of the secretions of Aphides and other insects.
Of Frost
Frost is, in most cases, nothing more than dew which has been frozen in the form of minute crystals, after being deposited on the surface of bodies cooled below the freezing point. The formation of frost, then, follows the same law as that of dew. Frost is distinguished from ice in not forming a smooth covering, but consisting of crystals of ice which hang sometimes loosely, sometimes fast, to the different objects, and resemble snow crystals in form. That frost is somewhat different in its origin which arises in winter, when, after a long period of cold, a warm wind follows, depositing watery vapor on all bodies without exception. This kind of frost also consists of ice crystals, with which projecting thin bodies, especially the stalks of plants, &c., are coated. It generally arises in clear nights, although sometimes when the sky is covered with clouds, and is especially frequent in polar regions, where the cordage of vessels is frequently covered with brilliantly white fringes. Fig. 59 exhibits a remarkable frost formation on the chiselled star of a tomb-stone. Figs. 60 and 61 represent the same on fallen leaves. Pl. 24, fig. 2, presents two trees trained along a wall, in which only those branches outside of the line, AB, are exposed to frost. To the second kind of frost belong the fine crystals which coat the thick walls of houses, when a returning warmth succeeds a period of continued great cold. An allied phenomenon, very interesting in its character, is exhibited in the formation of " ice flowers," or crystallizations of ice on the window panes of chambers. Some of these are shown in pl. 24, figs. 3–6. Fig. 1 presents a peculiar crystallization on the windows of a drug store, where the jars and bottles standing near the window were depicted on it in frost.
Of Fog
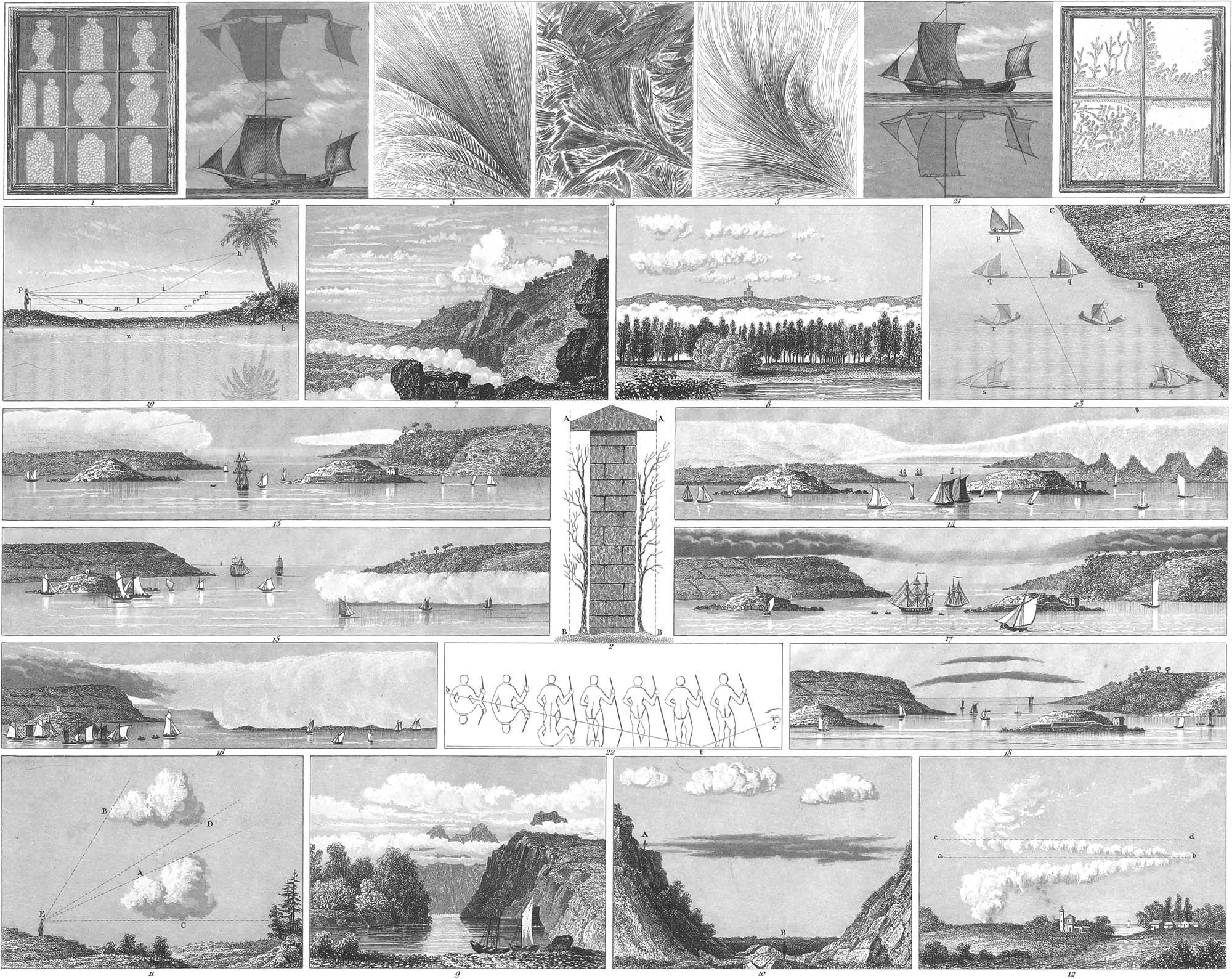
Fog may be produced in two ways; first, by the cooling of air filled with moisture to the dew point, the condensation taking place in the air itself, and impairing its transparency; secondly, by the diffusion of vapor arising from seas, lakes, rivers, or moist ground, in a cold atmosphere already near the point of saturation. It consists of minute vesicles of water filled with air. Fogs are very frequently seen over the surface of water in winter during calm and cold weather; also early in the morning during spring and autumn. Of a similar character is the cloud which forms over the surface of meadow or other moist lands. In all these cases the temperature of the air at the surface is less than that of the water or soil, the air itself being nearly saturated. Water cools much more slowly than air, owing to sinking of the superficial strata when cooled, their place being supplied by warmer portions. If, then, considerable bodies of water be of nearly the same temperature as the air, it will happen that during clear nights their temperature will be higher than that of the surrounding land. The air over the land will thus be cooler than that over the water. If the two (supposed to be near the point of saturation) be mixed, a precipitation of some of the water in the form of a fog will result. Pl. 24, fig. 7, is intended to illustrate the formation of fog at a certain place in England on a particular day. The temperature of the surface of the river was 56°, that of the air six feet above the water 47
Counting every day during which fogs are present for any length of time as a foggy day, we shall find that for one and the same place, the number of such days in different years is very nearly the same; not so, however, in different places. It has been observed that by far the greatest number of fogs experienced in London, Hamburg, Berlin, Stuttgard, and Munich, occurred in winter and autumn, while in Moscow foggy days are as numerous in summer as in winter.
Fogs are much rarer on level lands than in mountainous regions; on the dry plains of Asia and Africa they are almost entirely wanting. Next to mountains they are thickest on the shores of large bodies of water, and are more abundant as we approach the poles from the equator. They are especially prevalent in the Northern Atlantic in the neighborhood of Newfoundland, New Scotland, and Hudson’s Bay, all noted for their fogs. The reason of their occurrence in these places is the condensation of the vapor arising from the Gulf Stream by the colder air. It often happens that fogs are very thick on the decks of vessels, while the tops look out on a clear sky. The coast of California is almost continually veiled in fogs; even on the coast of Peru they last from four to five months at a time. Fogs are also frequent on the coast of Norway and of England. Beautiful exhibitions of fog occur on the English coast, particularly on the hills inclosing the harbor of Plymouth (see pl. 24, figs. 13–18). Indicating the hill to the left by A. the promontory in the centre with the tower by B, and the wooded hill by C, then in fig. 13 we see a strong condensation of vapor over A, observed about 5 P.M., of July 22, and only a slight one over B. Half an hour later A presented the same appearance, but the fog had increased over B, and covered a part of C. About P.M. the fog had increased over A and completely enveloped B; it had become shorter but higher over C. On the 2d of June, about 8
Isolated masses of fog are often observed on mountains, having only a few feet of diameter, and vanishing at a certain height of air. This generally occurs during rainy weather, and is usually a sign of its continuance.
The densest fogs occur over cities; and these, in addition to vesicles of water, appear composed of various exhalations. This, for example, applies to the dense fogs of London, Paris, and Amsterdam, which may therefore be called mixed fogs.
Besides the fog composed of vesicles of water, there is a dry fog, the principal compound of which is probably smoke or other exhalatory matter. Occasionally it occurs in great quantity, as in the years 1783 and 1847. Without diminishing the transparency of the air as much as is done by fogs, it dims the sun, and causes him to appear red and shorn of his beams. This phenomenon is most frequently observed in the Netherlands, in Northern France, and Germany; more rarely in England, and still more so in Southern and Eastern Europe. In the United States it is especially prevalent, particularly in autumn, about the time of what is called Indian summer. Natural philosophers are not agreed as to the cause and components of this dry fog or haze; according to some it is of electrical origin, according to others it consists of the smoke and exhalations of burning and decomposing substances, as also from volcanoes. The latter theory is probably the correct one. The occurrence of this fog in Germany unquestionably depends on the burning of turf or moors in Westphalia and East Friesland, especially in the moor regions of the coast of the North Sea, where from May to July the turf is dug up, dried, and burned for manure. The spontaneous burning over of extensive tracts of forest land would also contribute to a very great extent.
Of Clouds
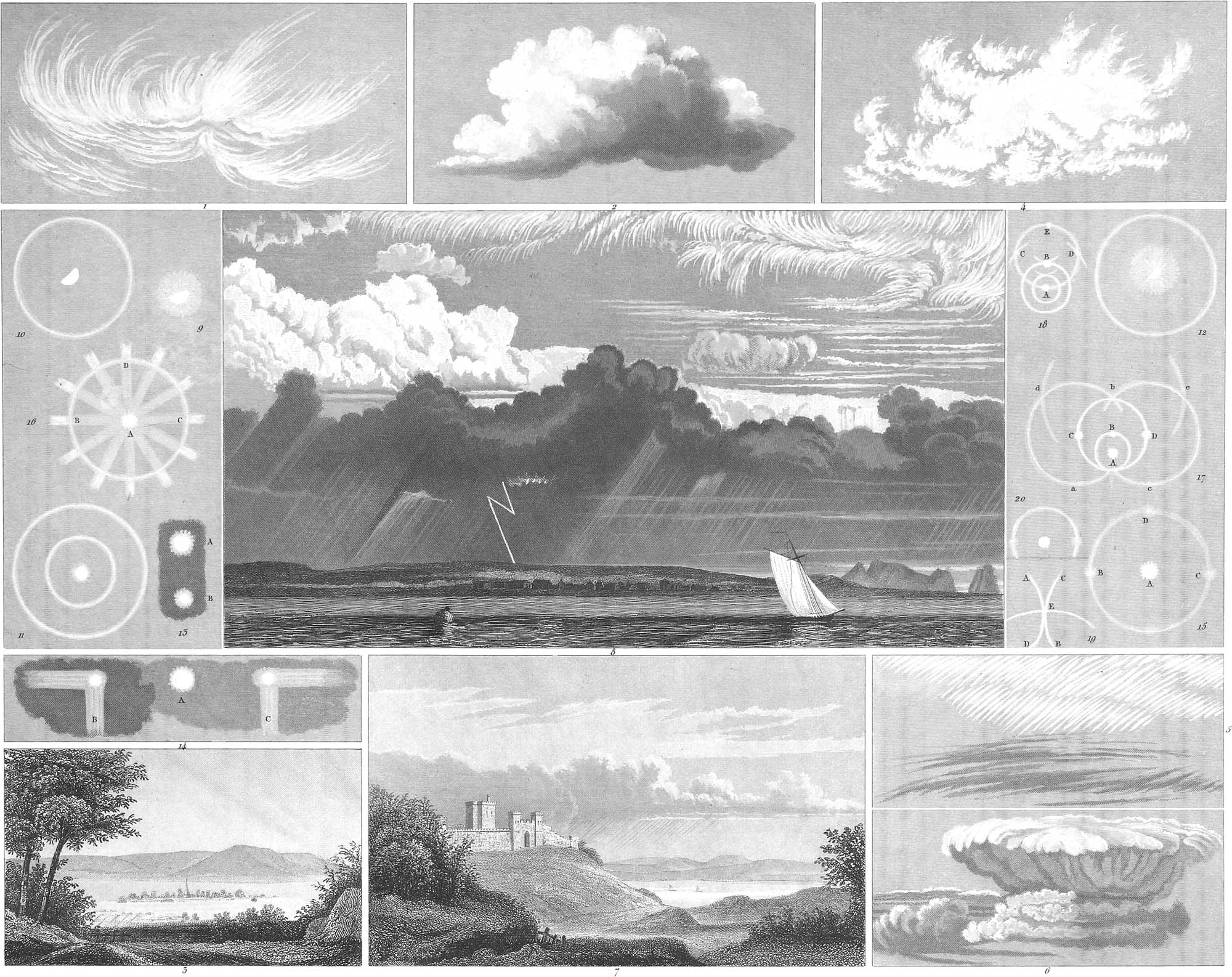
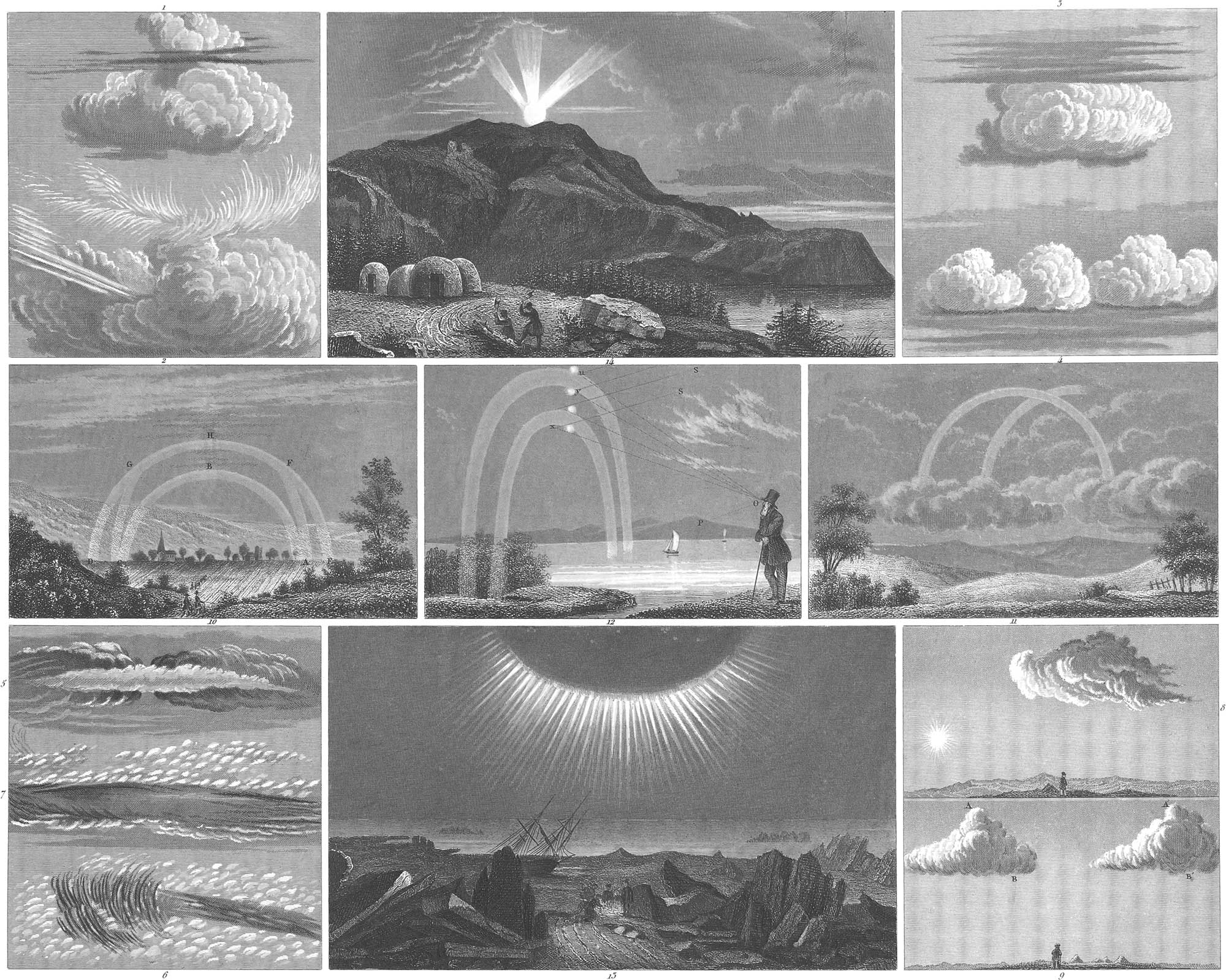
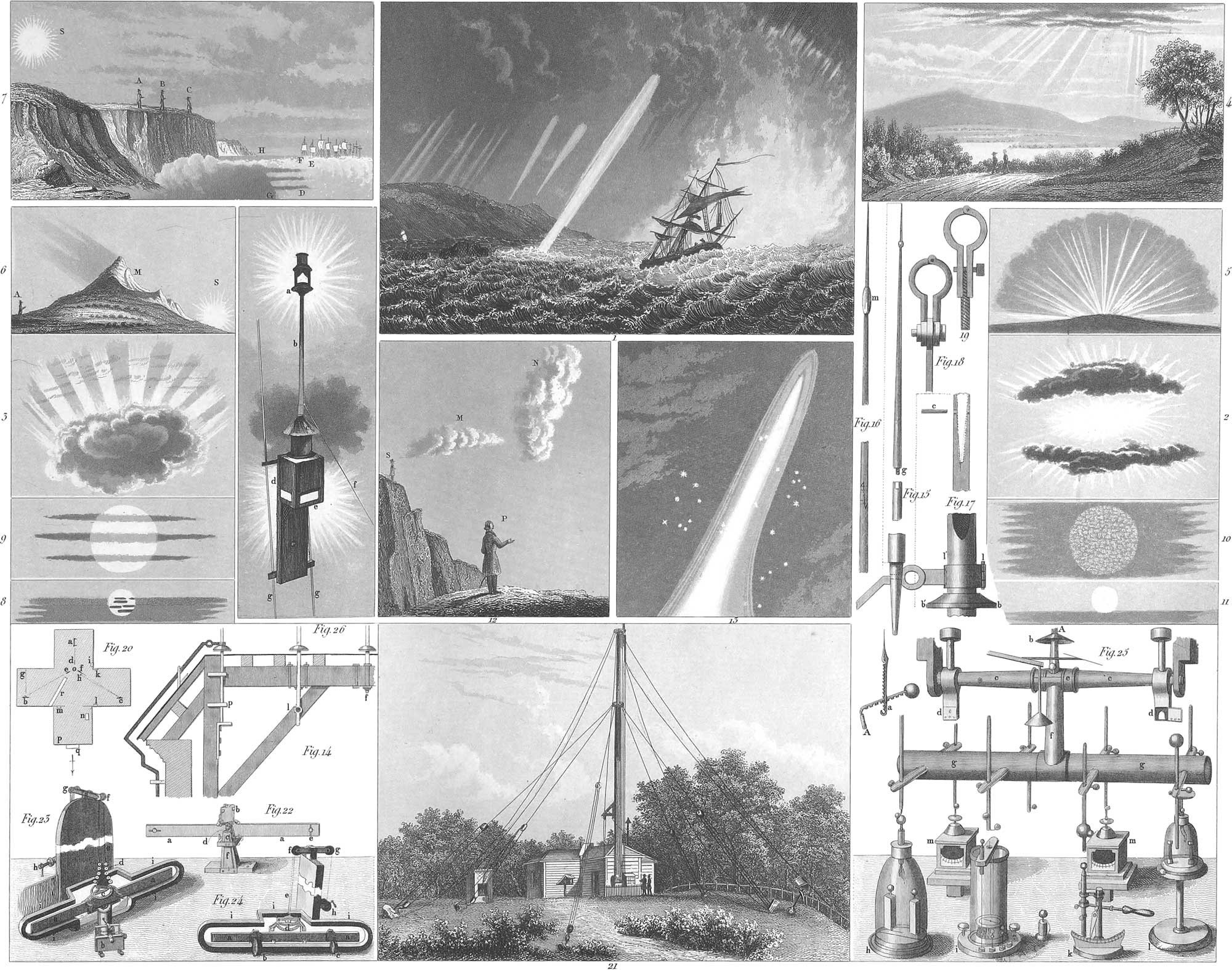
Cloud is nothing more than fog which has reached or been formed in the higher regions of the atmosphere. Although the water of which the cloud is formed is heavier than air, yet its disposal in the shape of hollow spheres diminishes very much its gravity; and even should it sink through the lighter strata of the air, it will ultimately come to a denser, warmer, and less saturated part of the atmosphere. Here the cloud will be dissolved at its lower face, while new fog will be condensed on it from above. Hence the nearer the atmosphere is to the point of saturation, the lower do the clouds sweep. Whenever the cloud gets into a current of air it is carried along with it.
The classification of clouds now almost universally adopted is that of Howard. He establishes three elementary and four secondary forms. The primary forms are: 1st. cirrus (pl. 25. fig. 1), consisting of light and feathery streaked filaments seen in clear weather; 2d. cumulus (fig. 2), composed of huge hemispherical masses, apparently resting on a horizontal base, occurring chiefly in summer, and presenting the appearance of heaps of snow; 3d. stratus (fig. 3), an extended horizontal layer of cloud, increasing from below, and appearing at times about sunset, of extraordinary brilliancy. The secondary or compound clouds are—1st. cirro-cumulus (fig. 4), forming the transition from cirrus to cumulus, and constituting the aggregations of small round white clouds, resembling sheep in a meadow; 2d. cirro-stratus (fig. 5), consisting of cirrus combined in horizontal or slightly inclined layers of considerable extent; 3d. cumulo-stratus (fig. 6), often giving to the horizon a bluish-black color, frequently seen to great perfection towards night of dry and windy winter weather; 4th. nimbus (fig. 7), or rain cloud. Fig. 8 exhibits cirrus and cumulus in the upper part above the thunder cloud. It must be readily understood that the precise reference of a cloud to one or the other of these modifications just described, must be sometimes a matter of great difficulty.
The sheep-like cirro-cumulus, when verging closely on cirrus, generally precede clear mornings and evenings, and are mostly indicative of continued good weather. Many philosophers, Kaemtz among the number, suppose the cirrus cloud which soars far above the others, frequently at a height of 20,000 feet above the earth, to consist not of vapor, but of particles of snow or ice. The cumuli are most abundantly seen in the horizon, and are of dazzling whiteness on their borders, and dark in the middle. Their appearance is frequently not unlike a snow-capped mountain. The stratus is often nothing other than a layer of fog or mist, and occurs at all heights, frequently of great extent. Cirro-stratus occurs in various forms and colors, being generally seen in morning and evening, and giving rise to the beautiful redness of the sky. According to Howard, that haze covering an otherwise clear sky as with a veil, and most frequently the forerunner of bad weather, belongs to this class. The thick rain-threatening clouds arising from the combination and expansion of cumulus belong to the cumulo-stratus.
The position of cloud is generally horizontal, it being only rarely that single clouds depend vertically, as in the case of wind and water spouts. The thickness of the different clouds is very various and difficult of determination. Peytier and Hoffard ascertained the thickness of cloud strata in the Pyrenees to be 1400 and 2600 feet in two successive days. The greatest observed thickness amounts to 5000 feet, although cases must occur where this is vastly exceeded.
The height of the clouds is much better known, although there are considerable difficulties in the way of ascertaining this elevation. Various methods of different degrees of merit have been proposed by Riccioli (for two observers at a known distance apart), Wrede (making use of the shadows of clouds), Kaemtz, Arago, and others. More recently Wartmann has proposed the use of the artificial horizon. According to Riccioli, the maximum height of the clouds is 25,000 feet. According to the measurements of Lambert, the minimum height is 7300, the maximum from 15,000 to 20,000 feet. Gay Lussac, after ascending in his balloon to a height of 21,000 feet, saw small clouds still at a considerable distance above him. According to Kaemtz, cumulus sweeps along at a height of between 3000 and 10,000 feet; cirrus between 10,000 and 24,000 feet; thunder-cloud between 1500 and 5000 feet. Pouillet instituted very exact measurements in 1840, during which he found clouds at an elevation of from 22,300 to 38,000 feet. In general we may assume that the thin cirrus cloud does not descend below 2000 or 3000 feet of elevation, while the thicker rainy clouds may come within a few hundred feet of the earth, although they may occur at much greater elevations. Clouds appear, furthermore, to attain a greater height in low than in high latitudes, the watery vapor being carried higher in the former than in the latter.
It is very difficult to ascertain the distance of a cloud from us, its apparent place being of not the slightest use in the determination. When the distance of a cloud is unknown, it becomes impossible to find out its actual size; and even the very shape is sometimes a matter of ambiguity. A change in the position of the cloud causes a change in its external appearance. This is shown in pl. 24, fig. 11, where the observer at E sees the same cloud at one time lower under the angle AEC, at another time higher, and evidently very differently, under the angle BED. Thus the same cloud might appear quite dissimilar from two different stations at the same instant of time. To an observer at A (fig. 10) the sky will appear furnished with clouds which are quite invisible to the one at B, the view being intercepted by a uniform stratum.
Clouds of very different character are often brought into contact by aerial currents or changes in the density of the atmosphere. Thus in pl. 26, fig. 1, a cirrus and a cumulus cloud are apparently brought into contact; in fig. 2 a cirrus appears resting on the summit of a cumulus; in fig. 3 a cumulus appears to have its summit cut off by a horizontal layer of cloud. Such contacts of clouds are partly real, partly only apparent. The apparent occur when two clouds lie in the same line of sight from an observer, although they may actually be quite widely separated. Thus to the mountain observer at S (pl. 27, fig. 12) the two clouds, M and N, appear to be in contact, while to the one stationed on the plain at P, their relation to each other is very different. In pl. 26, fig. 4, also, it is only apparently that a range of cumulus appears embedded in a dense black layer of cloud. Fig. 5 shows two clouds actually in contact, but likewise apparently combined by a long thin streak of cloud. Fig. 6 presents an apparent mixture of cirrus with light transparent fog, relieved against a mackarel-back sky. Fig. 7 shows an apparent contact of cirro-stratus with cirro-cumulus.
Clouds under different illuminations present very different appearances. Sometimes a cloud appears entirely in shadow; at another time its upper or lower border seems illuminated (pl. 26, figs. 8 and 9), A and B. As the shadows of clouds depend upon their different positions with reference to the sun, it is very evident that the same cloud will appear very differently in the morning and in the evening, in the north and in the south. If we assume that one mass of cloud, AB (fig. 9), stands in the south, and another, A′B′, of similar shape in the north, then to an observer between them the northern cloud, A′B′, will present its illuminated face, exhibiting only a small portion of the shadow, while the other cloud will appear entirely in shade.
In pl. 27, fig. 2 exhibits the appearance presented when the sun stands behind a cloud. Fig. 3 is an example of the diverging shadow of a layer of cumulus combined with cirrus. Fig. 6 illustrates the fact that objects on the earth, and especially mountains, can cast shadows into space.
About sunset diverging shadows of great beauty are often seen among clouds in the west. If these shadows are long enough to pass the zenith, they will converge to a point in the eastern sky opposite to the sun; the opposite phenomenon takes place at sunrise. A beautiful illustration of this, represented in fig. 5, was observed by Faraday in the Isle of Wight. Ten to twelve streaks of light and shadow were visible in the north-east, south-east, and south, apparently proceeding in a straight line from a point of the horizon between south and east. The atmosphere contained a light fog presenting but slight impediment to the sun’s rays. Clouds in the west prevented the transmission of light, and immense parallel shadows crossed the entire heavens, in an almost horizontal direction.
We must not omit to make particular mention of the beautiful coloring presented by the clouds, especially at time of sunrise and sunset. which we shall have further occasion to refer tp in treating of the morning dawn. As the clouds principally absorb the blue rays, they generally transmit the red, although we may have successive changes to yellow, orange, carmine, and purplish red. The situation of the cloud has great influence on its colors. In the immediate vicinity of the sun these are most brilliant. When directly in front of the sun they appear sometimes darker (pl. 27, fig. 8), and sometimes lighter and colored (fig. 9). This position of clouds just before the sun, gives rise at times to very interesting phenomena. A remarkable case was observed by Howard, where irregular streaks existed on a dark cloud, the intervals filled by clouds of a less dense but homogeneous texture. When the sun came behind the bright part of the cloud, the latter appeared suddenly covered by an irregular and rapidly moving network (fig. 10), although the great mass of the cloud exhibited no motion. No motion was perceptible when the sun came in contact with the dark streaks. Fig. 11 shows the apparent contact of the lower edge of the sun’s disk with an upper edge of a layer of cloud.
Of Rain
Rain is produced when, by the continued condensation of watery vapor, the separate vesicles unite into drops which are too heavy to float in the air, and consequently fall, increasing in size with the descent. To determine the amount of rain falling in any one part of the earth, we make use of an instrument called a rain gauge, and also ombrometer, udometer, hyetometer, pluviometer. &c. One of these is represented in pl. 23, fig. 64. It consists of a prismatic or cylindrical tin vessel, B, of five to seven inches in diameter, on which rests a second cylinder, open above, and with a funnel-shaped bottom perforated in the centre. Rain water falling on A passes through the aperture into the vessel B; this, however, is connected by the bent tube, C, with a glass tube, D, from which latter the height of the water in B can always be ascertained. If A and B have the same cross-section, the height of the water in B will express the depth to which the rain water would stand on the earth in a given time, provided that no evaporation and absorption took place. The rain gauge must be set in an open place, so that it may not receive water from any other source than the clouds. In temperate regions, however, the amount of rain which falls is sometimes only a few hundredths of an inch or line; we therefore advantageously use a graduated tube, whose cross-section is a known aliquot part of that of the rain gauge, and into which the water from the greater vessel flows or is poured. If, for example, the diameter of the tube is one third that of the rain gauge proper, the water will stand nine times higher in the former than in the latter, which gives us a much better opportunity of ascertaining the value of small amounts. Conical gauges are also employed, yielding excellent results in slight rains. Self-registering rain gauges have been constructed quite recently, some of considerable excellence.
Pl. 29, fig. 1, is intended to illustrate the different conditions with respect to rain presented by different portions of the earth. The annual fall generally diminishes as we recede from the sea, and increases with the height of the place above the sea; at one and the same place, however, the amount of rain decreases with the height above the ground. Thus Dalton observed that the amount of rain on the top of a high tower compared with that at the bottom was as 2 : 3 in summer, and as 1 : 2 in winter. From nine years’ observation at the observatory of Paris, it was found that the amount of rain on one terrace was about 0.116 less than on another twenty-seven metres below. The reason of this difference is, that fresh vapor is constantly being condensed on the drop in its descent, and consequently the drops must be largest just before reaching the ground. The nearer to the point of saturation the air happens to be, the more considerable is the difference just referred to; for this reason it is less at Paris in summer than in winter. If it be very great, long continued rainy weather is to be expected. This difference is not the same in all countries, and is less at Paris than in England. In warm countries, and in the warmer portion of the year, the rain is generally heavier than in cold countries and in the colder months. Between the tropics the rain sometimes falls to the depth of an inch in an hour, and Humboldt, in single instances in South America, observed from (bar to five inches in the same time. It is only very rarely that rain, to the amount of an inch in an hour, falls in higher latitudes.
On the continents of the torrid zone a rainy season of many months’ duration sets in about the time of greatest heat, the heavens being clear during the rest of the year. When the sun is in the zenith the rain is most violent and copious. The duration of the rainy season is generally three to five months. Near the equator, where the sun stands twice in the zenith, and, indeed, on days which are separated by several months, there are two wet seasons, either separated by a dry one, or exhibiting a maximum in the amount of rain. Thus Dutch Guiana possesses a great rainy season from April to June, and a lesser one from the middle of December to the middle of February. The rain drops of these and other tropical regions are remarkable for their magnitude, and produce a very unpleasant sensation on the naked skin. In the East Indies, where monsoons blow instead of trade winds, there are varying conditions of rain, the rainy season on the eastern coast occurring at the time of the north-east monsoon; that on the west coast at the time of the south-west monsoon. The rainy region of the calms does not possess any periodical rain. In some regions of the earth rains seldom, if ever, occur; among these are Egypt, the desert of Sahara, the high plains of Persia, northern Arabia, a part of Thibet, of Mongolia, &c. This is especially the case with the extensive arid and barren plains out of the tropics. The great heat and dryness of the countries is to be considered as the cause of this deprivation. Even on the high seas in those countries, where the trade winds blow regularly, rain is very rare, and the sky almost always clear. Rain is more abundant in the vicinity of mountain ranges on the warm plains of Asia and Africa. When, for instance, the vapors of the Mediterranean are carried south and east by a north wind, they become heated in their passage, and are thereby further removed from the condition of saturation. To this they again approximate by rolling up the slopes of the high mountains, and thereby coming into colder regions. Mountains everywhere exert a great influence on rain, the gathering of clouds about a mountain top being generally the precursor of approaching rain.
With regard to the condition of Europe in respect to rain, we find in Portugal a country where this is almost entirely absent during summer. North of the Pyrenees, however, copious showers occur in greater or less abundance throughout the whole year. To the north then of the Pyrenees and the Alps, we may distinguish two groups of climate, called by Kaemtz the middle European and the Swedish. In the former rain generally accompanies westerly winds, in the latter easterly, the westerly winds losing their water in crossing the crests of the Scandinavian mountains. St. Petersburgh and Moscow appear to lie on the confines of the two regions of climate, since there is a prevalent w 7 ind at neither of these places. In passing from the western coast of England to the interior of Europe, we shall find the annual amount of rain, as also the number of rainy days, to decrease continually. Calling the annual amount of rain at St. Petersburg unity or one, then on the coast of England this same amount is 2.1, in the interior of England 1.4, on the plains of Germany 1.2; although particular places may present considerable deviations from these proportions. Thus in western and southern England the annual fall of rain amounts to thirty-five inches, while in Kendall it is fifty, in Dover forty-four, in Bristol twenty-two inches; in middle and eastern England it is on an average a little over twenty-five, in Dumfries thirty-four, and in Glasgow only twenty inches. In France and Holland it is about twenty-four, and in Brussels onty eighteen inches. In Tegernsee it is forty-four, in Augsburg thirty-seven, in Carlsruhe, Ulm, and Gottingen, twenty-five, in Manheim and Ratisbon twenty-one, in Prague fifteen, in Würzburg fourteen, in Erfurt only twelve and a half inches. In St. Petersburg it is seventeen, in Abo twenty-four, in Buda sixteen, in Copenhagen seventeen, and in Stockholm nineteen inches. In the mountains of Norway, which have a very different climate, and are celebrated for their copious rains, the annual fall amounts to eighty-three inches. At Mahabuleshwar in India, on the western slope of the Ghauts, it is 283 inches, at Matouba on the Island of Guadaloupe 274, at Basseterre on the same island and at Anjarakandy in the East Indies 116, at Coimbra in Portugal 111, on the Island of Granada 105, in St. Domingo 101, at Havana 86, on the coast of Sierra Leone 81, at Bombay 73
The distribution of rain throughout the year varies much in different countries; in central Europe, which has a continental climate, summer rains have the preponderance, while in southern Europe this belongs to the winter and autumn. On the west coast of England the winter rains are more considerable than the summer, while in the interior this condition of things is just reversed. In the western parts of Scandinavia the winter rains are very copious, in Sweden they are almost entirely wanting, so that this country exhibits a transition from a sea to a continental climate so rapid as to have nowhere else its parallel. The rain of the warm seasons of the year is generally the most copious. Thus, taking the rain falling on a winter’s day as unity or one, the amount falling in summer will be indicated in the following mean quantities: in England, 1.07; western France, 1.03; central France, 1.57; Germany, 1.76; St. Petersburg, 2.17. The preponderance of the summer rain is thus seen to increase as we go east. In England and on the coast of France the average fall of rain on the autumnal rainy day amounts to two lines and three quarters for the former, and two lines and a quarter for the latter; in central France a summer’s rain is about 2.41, in Germany 2.33, and in St. Petersburgh 1.67 lines. The same laws, with respect to the predominance of coast rains in winter and central rains in summer, appear to apply also to other parts of the world.
Rain-water collected after a considerable amount has fallen, is almost chemically pure, and may be used in many cases instead of distilled water; yet it frequently contains slight amounts of certain mineral substances, especially chloride of sodium, generally combined with some lime. Traces of a somewhat greater quantity of salt are observed only on the coast and a slight distance from it, although most of the watery vapor contained in the atmosphere has ascended from the sea. Cases not rarely occur where various substances from the animal, vegetable, or mineral kingdom, either alone, or more frequently mingled with rain or snow, appear to have fallen from the sky. This is not the place for a closer investigation into these supposed showers of blood, fruits, sulphur, fishes, toads, stones, &c.; this much is certain, however, that in all cases, excepting in that of aerolites properly so called, these objects have been elevated by violent aerial currents, carried to a considerable distance, and then dropped on the abatement of the storm, provided, however, that a descent from the atmosphere has actually occurred.
Of Snow
The rain of higher latitudes is most frequently presented in the form of snow by the freezing of the rain-water in the air. In temperate countries this appears to freeze when the temperature is still some degrees above the freezing point, while in more southern climes it hardly takes place even when the thermometer sinks below 32° F. The shape of the particles of snow is exceedingly interesting; they occur generally as six-sided stars, consisting of needles combined at angles of 60° to 120° with each other. According to Scoresby. who has instituted the most careful observations on this subject, there are five principal forms of snow crystals: 1st. Crystals in the form of thin plates; these are most abundant. 2d. Surfaces or spherical nuclei with ramifying branches in different planes. 3d. Fine points or six-sided prisms. 4th. Six-sided pyramids; occurring but rarely. 5th. Prisms, tipped at one or both ends with a thin plate. Some of the most interesting figures observed by Scoresby are represented in pl. 23, figs. 4–23. The complete and regular crystals of snow appear only during severe cold. Should the temperature be several degrees above the freezing point, there will be a mixture of snow and rain; the crystals stick together, forming flakes of extraordinary size, which, however, are very irregular in shape, and soon melt.
In the torrid zone snow occurs only at great heights above the level of the sea, this elevation at the equator amounting to 11,000–12,000 feet. The highest mountain peaks, in all countries, if they reach above the snow line, will often have snow when rain is falling below them. In Europe the region of snow first commences in central Italy, while in Asia and America it descends much further south. The isothermal line of 59°F., which passes through Florence, may be taken as the commencement of the region where snow falls in the lowlands. At a mean latitude, and at a moderate height above the level of the sea, snow generally falls from an overclouded sky, when the weather becomes somewhat milder after severe cold, and at a temperature higher than the severest winter cold. It is an error, however, to suppose that it cannot snow during very severe cold. The latter occurrence is not rare in Germany, and Kaemtz observed snow to fall at Halle when the thermometer stood at from 5° to 1°F. After snow the weather sometimes becomes warmer, sometimes colder, more frequently the latter, and the ensuing cold is severe in proportion to the diminution of temperature during the snow-fall itself.
In Germany, and other countries nearly of the same latitude, snow generally falls in a quiet atmosphere; sometimes, however, and particularly in February, the falling of snow is accompanied by tempestuous weather. The wind in such cases frequently passes during the storm from west or south-west to north, and after the fall, cold weather with a clear sky sets in. On high mountains, and in mountainous regions, the falling of snow is often accompanied by winds which are not far behind the most violent storms in intensity, hence they are called snow storms. The snow storms of the Alps, so dangerous to travellers, are well known to all. At higher latitudes these storms occur at a less elevation, particularly in Norway and Kamtschatka, where they are called Purga. That such snow storms ought to be ranked with thunder storms is shown by the electrical condition of the atmosphere, and by the lightning which not rarely accompanies them.
The quantity of snow deposited differs with the year and country, being very variable for slight elevations above the level of the sea. More snow falls in valleys and woody districts than in the level land. The snow increases from the isothermal of 59°F. in the north, to the isothermal of 41°F. which passes by Drontheim; it then diminishes, since in the high northern latitudes the air is too cold to contain much moisture.
The quantity of water which may be obtained by melting snow differs greatly at times. During severe cold and northern or north-eastern winds, the snow is very loose and balls together with great difficulty, indicating the existence of little water. In general, the snow is lighter the more there is to be of it; hence the proverb, “much snow little water, little snow much water,” is well founded. According to De La Hire, water is 3
Snow water is generally pure, being only exceptionally mixed with foreign ingredients, for which reason it is of no especial use to vegetation, being rather prejudicial on account of the coldness. The beautiful red color of snow first observed by Saussure (1760) in the Alps, owes its redness to minute algæ (Protococcus nivalis). Green snow is caused by Protococcus viridis.
Of Hail
Hail, one of the most peculiar phenomena in meteorology, is divisible into two principal classes: 1st. Sleet, composed of round granules, generally not more than two and a half lines in thickness, always opake, and of snow-white color, occurring in wintery weather. 2d. Hail, properly so called, consisting of granules of spherical, paraboloidal, or pyriform shape, varying in size from a cherry-stone to a walnut. These have generally a point, opposite to which is a hemispherical segment, and in their centre is an opake nucleus of one half to two lines in diameter. This species occurs generally in summer, in connexion with thunder and lightning. The two kinds, however, according to Kaemtz, differ only in size. As a third and very rare species Arago considers those granules which never have a nucleus of snow, and differ from sleet of equal size by being transparent. These are unquestionably produced by the freezing of drops of rain in falling from a cloud into a stratum of colder air.
The form of true hailstones is very various; generally they are rounded, sometimes flattened or angular. Delcross supposes the most common form to be a three-sided spherical segment, produced by the shattering of larger spheres. Hailstones of different forms are represented in pl. 23, figs. 24–35. The internal structure is almost as various as the form; sometimes alternations of transparent and opake strata are Observed. The diameter of simple hailstones at a mean latitude, according to Muncke, is not over one and a half or one and three fourths inches, larger masses appearing to be produced by the aggregation of individual stones. Instances of hailstones, the size of hens’ eggs or larger, are not rare in some parts of the world. There are cases on record of vastly larger hailstones than those just mentioned; most of these, however, are of a very fabulous character. Thus in 1719 hailstones fell at Kremo weighing six pounds, and at Namur, in 1717, weighing eight pounds. According to Wallace, pieces of ice a foot thick fell in the Orkney Islands in 1680; in 1795 pieces of ice, six to eight inches long, and two fingers thick, fell in New Holland. According to public prints, a lump of ice fell in Hungary on the 28th of May, 1802, three feet long, three feet broad, and two feet thick, estimated to weigh 1100lbs. In the latter part of the reign of Tippoo Saib, a lump of ice the size of an elephant fell near Seringapatam. In all these cases the mass of ice most probably consisted of an aggregation of single lumps frozen together on the ground. It is only rarely that foreign substances have been found in hailstones. In 1755 these fell in Iceland containing sand and volcanic ashes; in Ireland, in 1821, hail with a metallic nucleus, recognised as sulphuret of iron; in 1824, in Siberia, hail containing octahedrons resembling auriferous pyrites. According to the earlier observations, small pieces of chaff are often found in hail.
Hail generally falls during the day, although the idea that it never falls by night is erroneous, there being well authenticated cases to the contrary. It is very probable that the rarity of night hail is only apparent, not real, and owing to the greater difficulty of observing such phenomena during the darkness. It has already been mentioned that the smaller hailstones generally fall in spring: in Germany in April, during that condition of the weather known as April weather. Short showers of cold rain then alternate with warm sunshine, and with the rain there fall either single hailstones, or the shower begins with single rain-drops followed by hail, the whole again concluding with rain. This alternation sometimes occurs in May and June, rarely in July, and perhaps never in August and September. Not unfrequently sleety hail is mixed with snow, either at the beginning, or more frequently towards the end of winter, in February or March. Fine granular hail frequently occurs on high mountains, where regular hailstones seldom are seen; thus on the higher Alps Saussure found twelve falls of the former to one of the latter.
The real hail storms belong to the summer season, and are accompanied by the severest thunder and lightning. They are therefore most frequent in June and July, rarer in May, August, and September, and seldom experienced in April and October. Storms of this kind generally arise after clear calm weather, accompanied by long continued oppressive sultriness. The hail clouds appear to sweep very low, with their edges jagged, and their lower faces presenting irregular projections, the parts yielding hail generally forming remarkable white streaks, the rest of the cloud being very dark. The barometer and the thermometer sink rapidly, the latter sometimes as much as 77° F. A peculiar rustling in the air announces near and heavy hail clouds; subsequently a darkness ensues not dissimilar to that produced by an eclipse of the sun. The hail itself lasts but a few minutes, with short interruptions, and generally accompanied by thunder and lightning, the duration being rarely over fifteen minutes, which, however, is often sufficient to cover the ground with a thick bed of ice. Hail storms move with great velocity; the one which occurred in France in 1788, so remarkable for its great extent, traversed the country at the rate of 38.8 Paris feet in a second, or forty geographical miles in an hour. The force with which the hail falls is very great, and, indeed, greater as the wind is more violent. Men are not seldom injured and even killed, a calamity which occurs quite frequently to small animals. Window-glass is very often broken, and even the roofs of houses and the branches of trees are at times unable to resist the terrible visitation. The injury produced in fields, gardens, &c., is often almost irreparable, as in the storm of 1788 in France, which devastated 1039 parishes, and caused a loss to the amount of more than twenty-five millions of francs. The quantity of hail which falls is rarely measurable with any degree of exactness, owing to the rapid melting produced by the accompanying rain, and the more elevated temperature of the air or ground. A depth of six inches is sometimes attained, although this very rarely occurs, at least in our latitude. A torrent of rain generally follows a fall of hail, lasting rarely more than half an hour. The area traversed by the hail is generally but small in width, often but a few hundred or thousand feet, rarely over a mile; the length of this area, again, is sometimes very great, amounting to over 400 miles in the storm just mentioned.
In the tropics hail rarely occurs excepting at great elevations; in the far north, again, large hail is very seldom seen. In general the tract of hail proper is confined to the region between 30° and 60° degrees of latitude, and to elevations under 6000 feet. Even within these limits there are countries where hail seldom or never occurs, as in some valleys of Switzerland, especially in the Valais, and in most of the valleys extending east and west. In the low lands at the foot of high mountains, hail is more abundant at a certain distance from the mountain than nearer or more remote. The same region is sometimes ravaged by hail storms for several years in succession, and afterwards again spared for a considerable time. From the comparisons of Kaemtz, who assumes but one kind of hail, there occur in France every year from ten to twenty hail storms (the most in spring, the fewest in summer), over five in Germany, five in Rome, and three in the interior of Europe.
Of the many propositions for preventing hail by the use of hail conductors none have yielded any practical results. The methods suggested may exert their influence in three ways: either by drawing off the electricity, by mechanical agitation of the strata, or by a chemically decomposing influence on the composition of the atmosphere. Hail conductors, or rods of the first kind, were proposed by Guenaut de Montbeillard in 1776. In 1820 La Postolle, and after him Thollard, recommended hail conductors of straw ropes, attached to pointed rods, or of straw ropes with a metallic wire interwoven. Curiously enough, these methods were much followed without the least benefit flowing from them. At the present day most meteorologists are agreed that there is no certain, or at least practical method for preventing the occurrence of hail storms.
On the Optical Phenomena of the Atmosphere
The air, although among the most transparent bodies in nature, is not perfectly so. Were this the case, its individual particles would reflect or scatter no light, and even by day the canopy of heaven would appear perfectly dark or black, with the exception of the space occupied by the sun and stars: sudden and total darkness would likewise ensue immediately on the setting of the sun. The general illumination of the heavens, and of objects not immediately reached by the direct solar rays, as also the gradual transition from daylight to the darkness of night, can only be explained on the supposition that the air does not transmit all the light, but reflects one part of the light traversing it, and absorbs another. The latter circumstance produces an enfeebling of the light, as we may readily perceive on examining remote terrestrial objects. In such an examination we find objects becoming more indistinct with increasing distance, vanishing at last altogether. The diminution of the angle of vision has of course something to do with this result, but not everything, since the effect thus produced varies at different times.
An instrument called the diaphanometer, invented by Saussure, is used to determine the transparency of the atmosphere. This savant painted black circles of different diameters on a white ground, and then removing from them, determined the distance at which they disappeared; this served as a measure of the transparency in question. In the higher regions of the atmosphere, this enfeebling of light is much less than at the surface of the earth, on account of the greater rarity of the strata of air. Watery vapor, and the smoke and dust carried aloft in various ways, exert great influence on the transparency of the air. Gaseous vapor appears to increase in a very high degree this transparency, as oil does that of paper. Nevertheless in opposition to this theory, the dry air in the interior of continents, as in Siberia, Persia, Africa, and Brazil, is remarkably transparent. Condensed vapor may diminish or even entirely destroy the clearness of the atmosphere.
The remarkable blue of the sky, or rather of the atmosphere, is not of the same tint in all places and at all times. In and about the zenith the heavens generally appear the darkest, decreasing thence to the horizon. This color is very dark blue when seen from high mountains. It is darker in the torrid zone than in higher latitudes; in Italy and Greece more so than in Germany; above the sea more than over the land in the same latitude. To compare the various shades of blue, and to determine them according to a given scale, Saussure invented the cyanometer. This consists of a circular plate, on whose periphery, white and black, and between the two, fifty-one different shades of blue are painted. The principle on which this scale is constructed depends upon the fact that the difference of color of two surfaces painted with different shades of the same color, vanishes entirely at a certain distance. Two blue surfaces differ, now, by one degree when the difference of their color is insensible at a given distance (according to Saussure, that at which a black circle of 1
Besides the blue color, the heavens frequently present at the rise or setting of the sun, a beautiful redness, the so-called morning and evening red, whose color changes into innumerable shades from yellow and bright red to dark red. The evening red (generally more abundant and brilliant than the morning) is most beautiful when the sky is of a very deep blue. The sun then at his setting appears very luminous, although not very red; even before sunset the whole horizon appears reddish yellow, this increasing until just as the sun is going out of sight: a remarkable reddening simultaneously presents itself in the eastern heavens, opposite to the sun, reaching its maximum as the sun goes down. Just after sunset, a light purple red is often seen shading the whole blue of the sky, and with the increasing descent of the great luminary the eastern heavens become dark, and there is often observed there a more or less evident segment of dark blue, whose highest point is directly opposite to the sun, and which is generally sharply defined (sometimes by a white or yellow border). The lustre of the western skies passes at the same time from the golden, more into the red; this portion, however, not extending very high. The evening red appears most, magnificent when, with a deep blue sky, there are some clouds in the west. Should these be cirro-stratus clouds, they appear before sunset as grey streaks with bright borders, the latter becoming afterwards golden yellow, and often fiery red. Clouds near to each other are often very differently tinted, some dark red, some yellow; this depending on a higher or lower position in the atmosphere.
This redness of the evening and morning sky has been generally explained by saying that the air transmits more readily the yellow and red rays, while it reflects more readily the blue. The sun’s rays having to traverse a considerable space through the atmosphere at his rising and setting, the air has an opportunity of acting to most advantage on his light, and of decomposing it, absorbing most of the blue. More recently, Forbes has attempted to show that this explanation cannot be correct, since the blue of the sky, strictly speaking, is not complementary to the evening red; the latter he explains by the watery vapor contained in the air, which, when perfectly gaseous, is quite transparent and colorless, but in its passage to its condition of condensation, transmits only the yellow and red rays.
The evening red and the morning grey are generally considered to be signs of fine weather: that the morning red is indicative of bad weather is by no means so universally correct. When the evening red is tinted with a soft purple red it certainly indicates continued fine weather, which is not the case with a whitish yellow, a very red, or a dull red evening sky.
Of Twilight
Closely connected with the phenomena of the morning and evening red stands the twilight, that gradual passage from daylight to darkness, and the reverse. Although the term twilight applies to both cases, yet the latter only is meant when we speak of the dawn. When the sun shines on the upper strata of the air, after ceasing to illumine the surface of the earth with his beams, a certain portion of the light is reflected towards the earth, thus producing a considerable degree of light. The beginning of morning or the end of evening twilight is marked by the disappearance or appearance of stars of the sixth magnitude. The depth at which the sun stands below the horizon at the beginning or end of twilight is differently estimated, varying from sixteen to twenty-four degrees, generally eighteen. As the sun’s orbit in higher latitudes is very slightly inclined to the horizon, twilight there lasts longer, while at the equator itself, where the sun’s orbit is perpendicular to the horizon, it is shortest, the sun occupying the least time in traversing the eighteen degrees just below the horizon. To find the duration of twilight at any place and day, it is only necessary to ascertain how much time is required by the sun in that part of its orbit to get eighteen degrees below the horizon. There will be two periods of shortest twilight in each year at every place. In estimating the actual and not the astronomical length of twilight, however, it is necessary to remember that the varying amount of moisture in the air will influence the reflection or dispersion of the solar rays very materially. Thus if the particles of vapor exist abundantly at a great elevation, and the lower strata of air be very transparent, twilight may last an uncommonly long time. Between the tropics twilight is always of very short duration; in Cumana, on the coast of Sierra Leone, at Paramatta, &c, it lasts only a few minutes; in Chili, during the dry season, only a quarter of an hour, although the time calculated, according to the preceding principles, would allow an hour and twelve minutes at the equator itself, even at the time of the equinoxes. The polar regions rejoice in very long twilights, at whose beginning and end the sun is probably thirty degrees below the horizon.
Various Phenomena of Atmospheric Refraction and Reflection
The twinkling of the stars, frequently more conspicuous at one time than at another, is produced by the agitation of vapor by aerial currents, and may justly be considered, when very remarkable, as a presage of wet weather; it depends upon the difference in the refraction of light in warm and cold, wet and dry air. It is sometimes accompanied by a change of color; in the fixed stars it is incomparably more evident than in the planets. Stars in the vicinity of the horizon sparkle more than those nearer the zenith, these frequently appearing nearly quiet. The twinkling is greatest when currents of unequal temperature move in contact, mixing partially; for this reason it is not the same in all regions, being visible during the dry season in the tropics only near the horizon, and but feebly there. In higher latitudes it becomes very striking during severe cold and a clear sky.
The so-called drawing of water is another phenomenon falling under this head. This (pl. 27, fig. 4) takes place when, while the sky is well covered with broken clouds, the sun stands behind a rain cloud and shines through openings in it. By the reflection of drops of water, particles of dust, &c., light streaks are presented, which, while apparently converging towards the sun, in reality are parallel. This appearance is seen more frequently in summer than in winter, oftener when the sun is low in the horizon than when he stands higher, and may generally be taken as indicative of approaching rain.
Rays proceeding from the sun rind other luminous bodies are refracted on their entrance into the atmosphere. As this, however, is composed of innumerable strata, increasing in density to the surface of the earth, these rays must be continually refracted, and thus move in curved paths which are concave to the earth. The consequence of this is, that unless a heavenly body is actually in the zenith, where no refraction can take place, its place, as seen by our eyes, will be higher than its true place. The amount of this displacement increases from the zenith to the horizon. Near the horizon it is half a degree, at an elevation of 45° it is one minute, and at 75° only one fourth of a minute. The sun (as also other luminaries) is thus seen in the morning before his actual appearance above the horizon, and in the evening after he has already set. The day, then, is lengthened by refraction, this lengthening amounting during the longest days to eight or nine minutes in Germany, and at higher latitudes to much more—to days and weeks in the polar regions. The same cause produces the oval form of the sun when just above the horizon, his lower limb being more refracted than the upper, his vertical diameter being thus abbreviated while the horizontal suffers no change; the result is the apparent elongation of his disk. A similar effect is produced on terrestrial objects, for which reason we distinguish a terrestrial from an astronomical refraction.
Refraction varies at different times, owing to its dependence on a variable condition of the atmosphere. For this reason the horizon does not appear always in the same place; it seems lower when the air at the surface of the ground is warmer and rarer than at an elevation of some feet; and higher when the ground is colder. Should the ground be strongly heated we shall observe a lively shaking or tremulous motion of objects, owing to the existence and combination of air strata of different densities and unequal refractive powers.
When the sun is very hot and the air calm, the lower strata of air, heated by the sun, often have a less density than those above them, without changing their position. In pl. 24, fig. 19, let ab be the horizontal surface of the earth, h an elevated point or object; let the eye of an observer be at p. He will see first a direct image of the object, h. in the direction ph, in which the rays, being only slightly deflected from a right line, will only produce irregularity in the outline of the image. Other rays, however, coming from h, follow the path hilmnp, since the ray, hi, which traverses strata c, c′, c″, c‴, of decreasing density, is continually refracted from the perpendicular, becoming more and more acute-angled to the horizon After traversing a sufficient number of these strata it ceases to be refracted any longer, and then it is reflected, reaching the eye in the direction mnp. The observer will thus see an inverted image of the object, h, in the direction pz.
Phenomena of this kind generally occur in hot countries, especially in deserts, as, for instance, in Egypt, where the French army was frequently bitterly deluded by this mirage. In Lower Egypt the ground forms a vast and perfectly horizontal plain, exposed to the overflow of the Nile. The villages are there built on small hills on the bank of the river, or at some distance from it towards the desert. The air is generally calm and pure; early in the morning and in the evening, therefore, objects appear in the natural positions and distances. But when the ground becomes heated by the ascending sun, and with it the lower strata of the air, a tremulous motion will be observed in the distance, such as is seen during a hot and calm summer day, or such as takes place sometimes over a heated stove. Should the air be perfectly calm, so that these inferior strata are not disturbed in the least, a direct image of all the villages and other elevated objects will be seen in the distance, and under them a reflected image, just as if they were situated on the banks of a quiet lake. The ground thus appears to be covered with water, in which the hills stand like islands. On approaching nearer, the apparent outline of the water recedes, always keeping at a certain distance from the observer. This phenomenon is frequently referred to by Arabian authors, as also in the Koran, under the name of Serab. It is likewise known in Middle India, where it is called Chittram, Dessasur, &c. In many cases, instead of an inverted image under the distant objects, only a bright streak of light is perceived, this image being too small to be perceptible. Thus we sometimes see the ascending sun apparently floating in the air, especially when the eye is near the ground.
Even at sea, and under quite different circumstances (namely, when the ground is colder than the contiguous strata), similar phenomena present themselves. In general we may distinguish three different classes of these phenomena. To the first belong those cases where distant objects, which generally are concealed by the curvature of the earth, become visible for a short time. This occurs both by land and sea, especially in the Northern Ocean. To the second class belong the cases in which distant objects appear surrounded by water, a phenomenon occurring in extended plains, and not confined to warm countries, but even found in the Steppes of Russia. In the third class are placed those complicated appearances in which the object appears double, and sometimes even three-fold; here an upward or downward reflection is distinguishable, according as the secondary inverted image appears above or below the primary. Thus, not unfrequently, vessels just visible in the horizon are seen double, a second inverted image being observed above an upright one, the mast tops of the two pointing towards each other (pl. 24, fig. 20). Again, in the case of a vessel whose masts just loom above the horizon, we may see two images, one erect, and beneath this another inverted (fig. 21). The latter (downward) reflection rarely occurs on land. Professor Vince once saw at Ramsgate, on a sultry evening, after a very hot day, the mast of a vessel projecting above the horizon, at some distance above it the image of the whole ship inverted, and above that again an erect image, the two latter separated by a strip of sea. When the vessel approached, the upright image vanished first, then the strip of sea, and finally the inverted image.
The time-renowned phenomenon of the Fata Morgana, observed on the coast of Calabria, and especially at Reggio, belongs in this place. At a certain time houses, palaces, colonnades, groves, hedges, &c., are seen above the sea, the whole being but the aerial reflection of the city of Messina and its environs. Most of the earlier descriptions of this wonder are exceedingly exaggerated and highflown.
Monge, Vince, and Biot, have, more than any other philosophers, been occupied in investigating these interesting and remarkable phenomena. The latter has shown how, under certain circumstances, a line, btc (pl. 24, fig. 22), may be supposed drawn from a distant point, b, beneath which all objects will be invisible to an observer at c, while of all objects above this line two images will be seen, one direct above the line, and another inverted below. Thus a man walking from the observer would successively present the appearances of fig. 22.
Sometimes the unusual or secondary images lie neither above nor below the primary, but at one side of it. An apparition of this kind was seen by Soret and Jurine, Sept. 17, 1820, on the Lake of Geneva (pl. 24, fig. 23). While standing in the second story of a house on the bank, they looked through a telescope in the direction gp, at a ship, p. which was two miles off and sailing towards Geneva. While the vessel came successively to cqrs, they noticed to the left hand distinct images, q′, r′, s′, which separated more and more from the direct or primary image of the vessel with increasing approximation. The air over the lake on the eastern bank, ABC, at that time had been a good while in the shadow cast by the mountains of Savoy, while the left, side was already heated by the sun. The plane of separation between the cold and warm air was thus vertical for a moderate height above the water. The sailing of the vessel just along the confines of the two regions produced the phenomenon in question.
On the Rainbow
Of all the optical phenomena of the atmosphere the rainbow is perhaps the most beautiful. It is seen when a spectator with his back to the sun looks in the opposite direction towards a shower of rain proceeding from an illuminated cloud. Two different bows are generally seen, one above the other, with their colors in the reverse order; the lower of the two is generally most brilliant. Suppose a straight line, OP (pl. 26, fig. 12), drawn through the sun and the eye of the observer, and a vertical plane passed through this line. Draw through O a line Ox, so that the angle POx amounts to 42°, then rain-drops which lie in the direction Ox will send colored rays to the eye, and as this is the case with all drops which lie in the surface of a cone formed by rotating the line Ox about OP, the eye will see a light circle of about 42° of radius. The centre of the circle lies where a line produced from the sun through the eye of the observer cuts the opposite sky, or where the shadow of the observer’s head would fall. The light of the sun entering the drops of water from above is refracted to the opposite internal surface, thence reflected to the lower part of the drop, and emerging reaches the eye of the observer, if he be at the proper angular distance. The light in these successive refractions and reflections becomes decomposed, and as each color has a different refractive power, it follows, that to see all the colors (violet to red) from the same drop it will be necessary to take up successive positions, so that the angle POx; may range from 40° 17′ to 42° 2′. If, however, as is actually the case, we have a series of drops, one above the other, and as the angle made between the axis of the box and the line from the eye to the individual drops, amounts to from 40° 17′ to 42° 2′, we shall, without changing our position, observe all these colors. The breadth of the arc is about 2°, the colors fading one into the other, and succeeding in the order of the spectrum, the red being uppermost. When the sun is veiled by mists or clouds the bow may appear simply as a light colored arc. The nearer to the horizon the sun stands the greater is the arc described; when the sun is in the horizon the bow is a semicircle. An observer under favorable circumstances may, from an elevated position, see more than a semicircle, and completely circular bows can often be observed in the spray from fountains, waterfalls, &c. When the sun is 42° above the horizon no bow will be visible.
Besides the primary bow just described, a secondary bow may almost always be observed outside of the first, but still concentric with it, and with the colors in the reverse order. The red is here the inner color, and the violet the outer. The colored bands are broader but weaker than the corresponding ones of the primary bow. This secondary bow is produced by the colored rays from drops at such an elevation that they just reach the eye after undergoing two refractions and two reflections, while in the first case there are two refractions and but one reflection. The same drop cannot furnish at a given instant to the same position of the observer colors belonging to both bows, although it may in different stages of descent, or to different observers. The angular position of the second bow is between 50° 59′ and 54° 9′, with respect to the axis or the line from the sun through the eye to the spectator; there may be more than two bows resulting from rays that have undergone more than two reflections, but the light becomes finally so feeble as to render it impossible in ordinary cases to distinguish them.
It is evident from the preceding principles that a rainbow may appear in the west in the morning, in the east in the evening, and in the north at noon; never in the south. Also, that every observer sees his own rainbow, and that this changes for him with every successive instant. A drop of water can only play its part to a fixed observer who sees two bows during two periods of time, the first in passing the limits of the upper bow, the second in passing across the second.
Sometimes other colors are added to the violet of the primary bow, as, for instance, a second green and second violet; red also is sometimes perceived. This same repetition of colors may also be observed occasionally on secondary bows. The cause of these irregular colors is not yet satisfactorily explained.
Some different rainbow formations are exhibited in pl. 26, figs. 10 and 11. Should the image of the sun be reflected from the surface of extended calm water into a rain cloud, this reflected solar image, for an observer sufficiently near the water, may produce the same effect as a sun standing below the horizon, and also producing a bow, which then regularly intersects the former. The manner in which this intersection is performed depends upon the elevation of the sun. Sometimes four rainbows are seen, where both the sun and its image produce two bows each. The reason that this phenomenon is not visible on the sea is, that the surface is rarely smooth enough, and the image of the sun in the waves is generally too indefinite.
A rainbow appearance is sometimes seen in the spray of the sea, or in the dew-drops of a meadow, where the curve is generally of an elliptic, parabolic, or hyperbolic shape. These bows, as also those seen in the mist of waterfalls, &c, are produced by the same causes as actual rainbows.
Lunar rainbows occur but rarely; sometimes they are colored (as at Forchheim near Freyberg in Saxony, November 22, 1847), but more generally white or yellowish, the feebleness of the moon’s light not permitting the colors to be distinguished.
Of Halos and Parhelia
An additional class of very complicated phenomena, likewise dependent on atmospheric reflection and refraction, is presented by halos and parhelia. We must distinguish two kinds of halo, lesser and greater, which, in appearance as well as in constitution, are completely different. The lesser halo is a colored ring a few degrees in diameter, seen around the moon, more rarely around the sun, when the sky is covered by a pale veil of very thin cloud (pl. 25, fig. 9). The red predominates in these rings, and sometimes several concentric ones are observed separated by interspaces, in which green may be detected. The dazzling action of his rays is the principal reason why this form of halo is seldom noticed around the sun. They may, however, be very frequently detected by assuming such a position as enables us, without looking directly at the sun, to see the contiguous portions of the heavens, or by observing the reflection of the sun in still water, or in a plate of glass blackened at the back. In June, 1692, Newton distinguished three different series of rings at one time: in the first the succession of color, commencing nearest the sun, was blue, white, and red; in the second purple, blue, green, bright yellow, and red; in the third, blue and red. The diameters of the three red circles were five, nine and a half, and twelve degrees. The phenomenon is very rare in the perfect form; in general, however, it has great similarity to the glory observed on looking at the flame of a candle through a glass plate on which lycopodium has been strewed. According to Fraunhofer, the phenomenon of these halos is produced by the refraction of light, by the vesicles of vapor in the air, as also by the interference of light.
When the clouds are not so dense as entirely to intercept the passage of rays from the sun, they all. excepting the cirrus, exhibit traces of colored rings. This phenomenon, although very frequent, is not always of equal beauty and distinctness; to observe it we may make use of the blackened glass plate already referred to. White clouds in the vicinity of the sun, with their edges parallel to the horizon, do not exhibit very well defined rings, but rather lively prismatic colors in the shape of streaks, ten and more degrees from the sun, and parallel to the horizon; these are generally green in the middle and surrounded by a red border.
The so-called halo of glory, seen about the human shadow when cast on the grass, or upon a surface curved with dew by a declining sun, is a curious appearance produced by the reflection of light from the dew-drops, or from the cylindrical smooth stems of the straw or grass; as the entire lustre depends on the position of the eye, the sun, and the reflecting surface, each one sees only the glory about the shadow of his own head, that of others, even though very near, being invisible.
Among halos is to be counted the appearance produced where the shadow of the observer is cast on a cloud, his head appearing surrounded by colored rings. The first observation of the kind was made by Bouguer in the Cordilleras in the middle of the last century. He and his companions, standing on the top of a high mountain, about sunrise, saw each one his own shadow depicted on an opposite cloud, the head surrounded by from three to four brilliantly colored concentric rings, the red being outside, and the other colors following in the order of the spectrum. Around these rings was another of a white color, and about 67° in diameter. Scoresby, in his arctic voyage, frequently observed this interesting phenomenon, which, according to him, always appears when sunshine and cloud co-exist, a circumstance which often occurs in the polar regions, the thin fog which there rests on the surface of the sea extending to a height of from 150 to 180 feet. An observer at the masthead of a vessel discerns his shadow in the fog opposite to the sun, the head surrounded by four or five colored rings. Howard and several companions once observed their shadows, and that of the rock on which they stood, depicted on a cloud or fog bank sweeping below them (pl. 27, fig. 7). This phenomenon is very common in the Alps, particularly on the Rigi and on the Brocken, where it is called the Spectre of the Brocken.
The larger circles of twenty-two to twenty-three, and forty-six to forty-seven degrees’ radius, sometimes seen about the sun and moon, are of entirely different character from those previously described. The colors of the smaller ring are generally much less lively than those of the rainbow, red, however, being recognisable on the inner edge, while the outer is very indistinct; the colors of the larger circle, which is much the rarer, exhibit more depth and purity. Pl. 25, fig. 10, represents a simple, fig. 11 a double halo of this character; fig. 12 is a simple halo combined with a colored ring or true halo. Halos about the sun and the moon are very abundant, but are generally appreciable only by means of the blackened glass plate; Kaemtz supposed that at least sixty were visible in Germany each year. The general. conditions of formation are pretty much the same in the two kinds of halos, the lesser and greater, the principal difference being, according to Kaemtz, that while cumulus cloud gives rise to the former, cirrus or very light cirro-stratus produces the latter. The two are never seen in the same cloud; when they do appear conjointly, the lesser is formed in a secondary cloud of fog.
Sometimes halos are accompanied by other phenomena, namely, by circles and arcs which pass through the sun, or by arcs intersecting each other, and exhibiting the so-called mock suns and mock moons, or sun and moon dogs, more scientifically known as parhelia (pl. 25, fig. 13), AB. The simplest case is where, the sun or the moon being low in the sky, a portion of a vertical circle in the shape of a column stands directly over the luminary (rarely under it). A white circle, moreover, frequently passes through the sun, often encircling the whole heavens, and parallel to the horizon, the breadth being nearly equal to the diameter of the sun. Should there be then a vertical arc above and below the sun, the latter will stand in the middle of a cross, a phenomenon but rarely observed. The mock suns appear most generally where the inner ring and the horizontal circle intersect each other, and are themselves frequently seen when the circles are invisible. They have the color of the inner ring, but are usually provided on the outside with a long shining horizontal train. The higher the sun, the more do the mock suns lie outside the intersection of the circles, and at a considerable elevation of the sun, two mock suns on each side are sometimes visible. Sun dogs sometimes occur at a distance of ninety degrees from the sun. Rarer phenomena are, tangent circles at the highest and lowest parts of the ring; tangent arcs at a distance of sixty degrees from the lowest part of the ring; a sun opposite to the true sun, and at an equal height with it; &c. (figs. 14–20). The phenomenon observed on June 29, 1790, by Lowitz in St. Petersburg, is to be considered as normal in its character. It lasted for five hours in an atmosphere filled with vapor; but cases of this perfection rarely occur. The most important individual parts of this celebrated illustration of parhelia were: 1st, a ring round the sun of about twenty-two degrees’ radius, red within and bluish without; 2d, a second colored ring about the sun of twice the radius of the preceding; 3d, a white horizontal circle passing through the sun and encompassing the heavens; 4th, five mock suns on this circle—two of them colored with long brilliant trains a little outside of the lesser circle, two white at a distance of ninety degrees from the sun, and one white and pale directly opposite to the sun; 5th, a lively lustre at the uppermost point of the inner ring, where at times a contact arc was seen; 6th, an arc convex to the sun at the lowest point of the ring just mentioned; 7th, a colored arc, also convex to the sun, at the highest point of the greater ring; 8th, two pale arcs passing through the parhelia and the upper part of the inner ring; 9th, two colored circles touching the outer ring, whose points of tangency were about sixty degrees from the lowest point of the former ring.
All these phenomena are explicable very satisfactorily by the refraction of light passing through prismatic crystals of ice, or snow crystals floating in the air. In proof of this, the appearances in question are most numerous in winter and in the colder countries (especially near the poles). It is a little remarkable, on this hypothesis, that they should occur in summer; but even then particles of ice may float at a great height in the air. Upward and downward currents of air are unquestionably of influence, since, after south winds have saturated the air with moisture, an ascending current carries this to such a height as immediately to freeze the moisture condensed by the cold; or a descending cold current may produce the same effect on the moisture of an inferior current. In this way we explain the very rare occurrence of halos in the torrid zone.
Of the Zodiacal Light
About the time of the vernal or autumnal equinox, and shortly before the rising or after the setting of the sun, there is seen a whitish streak of light, tending in the direction of the ecliptic or of the zodiac (whence the name), and running out above into a point. This is called the zodiacal light (pl. 27, fig. 13), which in spring is seen more frequently in the western evening sky, and in autumn early in the morning of the eastern heavens. The light of this object is generally much feebler than that of the milky way, being brightest in the neighborhood of the sun, feebler towards the point, and never so bright as not to allow the lesser stars to shine through it. The shape of the zodiacal light is that of a pyramid or cone, whose base rests on the sun; more accurately, that of a very eccentric ellipse, whose variable major axis passes through the sun, and is at least five times as long as the minor. The apex seems sometimes to run out into two straight lines which form an angle of 10°–26°; the cone, therefore, appears truncated. The axis falls nearly in the plane of the sun’s equator. The length, measured from the sun, amounts generally to not over 43°; sometimes, however, to 100°, and in single cases in the torrid zone even to 120°. The greatest breadth in the vicinity of the horizon varies from 8° to 30°.
In the torrid zone, where the zodiacal light stands almost perpendicularly to the horizon, it is not only more frequently seen, but is far more brilliant and remarkable than in higher latitudes. Even in the island of Guadaloupe it may be seen at any time of the year with a clear sky, while in our own latitude it is visible only in spring and autumn. Humboldt observed it on the Andes, and on the plains of Venezuela, as also at sea, more luminous than the milky way in the constellation Sagittarius, and the exhibition was more brilliant when the vaporous exhalations were projected against the light. At sea, between 10° and 14° N. L., he observed it for several weeks in summer in great beauty, and presenting a splendid play of colors : becoming visible an hour after sunset, it became weaker towards ten o’clock, and vanished almost entirely about midnight. He was especially struck by the varying brightness of its lustre: after being at its brightest, it would in a few minutes after become remarkably enfeebled, and in particular cases he thought he observed a kind of flickering and waving of the light. Our figure (pl. 27, fig. 13) represents the zodiacal light as observed by Horner, in the Atlantic ocean, N. L. 28°, on the night of Dec. 13, 1803. At that time it was visible as a reddish lustre in the evening twilight, of fifteen degrees in height; subsequently it extended more and more even to the zenith, near which it was about four degrees broad, the whole forming a triangle, whose base resting on the horizon was from eight to ten degrees in length.
The true character of this remarkable phenomenon is veiled in the greatest obscurity. Dominic Cassini, and, after him, Laplace, Schubert, and Poisson, assumed a ring encompassing the sun and forming the orbitual path of an innumerable host of small planetary bodies revolving around the sun. According to Fatio de Duillier, the zodiacal light bears a great resemblance to that of the tail of a comet; according to Euler it is identical with this latter. Mairan, with most of his followers, supposed it to be the sun’s atmosphere; Hutton referred it to the agency of electricity; Thomas Young derived it from a highly rarefied atmosphere of light revolving around the sun with a far greater rapidity than the earth. Regner, however, showed that there could be no luminous atmosphere around the sun, else it would be visible in total eclipses of the sun: he supposed the zodiacal light to be nothing else than light attracted and condensed by the illuminated hemisphere of our earth, and thus made visible at night. All these hypotheses are more or less untenable, particularly that which supposes the zodiacal light to be the luminous atmosphere of the sun, since, according to mechanical laws, this cannot be flattened more than in the ratio of 2 : 3, the greater axis being thus to the minor as 3 : 2. According to this computation it could not extend to more than
On the Fiery Phenomena of the Air
Excluding the electric and magnetic meteors, namely, lightning and the aurora, there remain to be considered, under this head, various phenomena more or less puzzling in their character. The principal of these are the ignis fatuus, the shooting stars, and the balls of fire; the two latter probably identical in their nature.
The ignis fatuus, also known as Will-o’-the wisp and Jack-o’-lantern, is the faint light or flame, generally about the size of that of a taper, which is occasionally seen to hover over the earth at a certain height in the atmosphere, and flickering here and there, sometimes vanishing almost entirely. It is at times accompanied by a slight smell of sulphur. These are most numerous in churchvards. marshy places, and other localities where dead animals exist, this being especially the case in warm countries during summer and the beginning of autumn, just after sunset. Reliable observations by scientific men on this phenomenon are very few in number, owing to the rarity of its occurrence. The distinguished astronomer, Bessel, while travelling in a boat to Bremen on the 2d of Dec. 1807, saw, during the very dark and rainy night, several hundreds of these lights over a piece of ploughed moorland. They were mostly of a bluish color, without much motion. In many parts of Spain and Italy, especially about Bologna, and on some dry hills near Nizza, great flames are sometimes seen to ascend from the earth to a height of twelve feet. They vanish suddenly, again become enkindled, and appear to be extinguished neither by wind nor rain, changing their places with considerable rapidity. There is no doubt that many of the accounts we have of the ignis fatuus, especially those of ancient writers, are highly tinctured with superstition and fear, to which we may also ascribe the idea that on approaching the light it always recedes and leads its follower astray.
According to Yolta, the real ignis fatuus consists of carburetted hydrogen gas inflamed by electricity. Gehler supposed it to be due to a phosphorescent matter generated by putrefaction. Parrot considered it as a marsh gas (probably a mixture of phosphuretted hydrogen and other gases) inflamed by the atmosphere. Berzelius asserts, however, that this theory is untenable, since the gases mix very rapidly, and the peculiar unpleasant smell of the gas just mentioned would be readily perceptible where such meteors occur. Muncke believed that in most cases hydrogen containing phosphorus in solution, if not phosphuretted hydrogen itself, must be considered as the cause of the phenomenon, but that some accounts and appearances are attributable to the phosphorescence of decaying animal or vegetable matter. In other cases, especially in warm countries, it is exceedingly probable that luminous insects, like the firefly, the glow-worm, &c, have given rise to the appearance in question.
A phenomenon of much more frequent occurrence is presented by the shooting stars. These luminous bodies, like stars, seem to glide rapidly across the heavens. As the subject has already been referred to under the head of astronomy, it will here be necessary to make only a few supplementary remarks.
The elevation of the shooting stars, that is, at the beginning and end of their being visible, is very various, ranging, according to the observations and measurements of Bensenberg and Brandes, from four to thirty-five miles. A series of observations made in 1823, on one hundred shooting stars, gave 4 of 1–3 miles, 15 of 3–6 miles, 22 of 6–10 miles, 35 of 10–15 miles, 13 of 15–20 miles, 3 of about 30 miles, 1 of 45–46 miles, 1 of about 60 miles, and 1 of over 100 miles. Olbers, however, considered all determinations of over thirty miles as highly problematical. Among thirty-six of the hundred just referred to, their observers estimated that twenty-six went downwards, nine upwards (the angle of inclination from 6°–68° to the horizon), and one horizontally. The orbits of most had a westerly direction, opposite to the motion of our earth in space. The average velocity of motion is from twenty to thirty-nine miles per second, more than twice as great as that of our earth in space.
The shooting stars fall either singly or in showers. The latter occur periodically, appearing most conspicuously from the 9th to the 14th of August, and from the 12th to the 14th of November. The unparalleled fall of stars which occurred in North America during the night of November 13, 1833, in which at least 240,000 fell in nine hours, first induced Olmsted and others to suppose that a certain connexion existed between these showers and certain days of the year. The fall of meteors observed at Cumana by Humboldt and Bonpland on the 12th of November, 1799, and by others in the United States, in Greenland, and in Germany, was called to mind, as also the showers in October of 902, 1202, and 1366; on the 9th and 10th of November, 1787, in Southern Germany; on the 12th and 13th of November, 1822, in Potsdam; on the 13th of November, 1831, on the Spanish coast; and on the 12th and 13th of November, 1832, in England, France, Switzerland, Germany, Belgium, and Russia. Since then the November meteors have occurred regularly about the same time of the year. With respect to the shower of 1833, Olmsted has shown that all the meteors radiated from the same point in the heavens, this point lying in the constellation Leo. According to Encke’s calculation from observations made in the United States at that time, all the stars proceeded from that point in space towards which the earth’s motion was directed at the time. Olbers thinks it exceedingly probable that a recurrence of the great shower may be looked for every thirty-four years.
A scarcely less regular meteoric shower takes place from the 9th to the 14th of August. Being observed about St. Lawrence’s day, it is sometimes called the shower of St. Lawrence. Muschenbroek, in 1762, first called attention to the abundance of these meteors in August; but Quetelet, Benzenberg, and Olbers, have more particularly determined the periodic return of the phenomenon. Other periodic showers have been indicated as occurring in April, towards the end of November, and from the 6th to the 12th of December.
Simultaneously with the shooting stars of November, 1833, were seen great balls of fire, and there is now no doubt that both are essentially identical. A common peculiarity of all fire balls is, that after being seen for a short time, they burst with a loud noise and the evolution of smoke; other than this, they are quite different at times. Sometimes they exceed in size the apparent diameter of the moon, and shine with such lustre as even in tropical countries to be visible in bright day; their apparent diameter, however, is no doubt greatly overestimated. Some of the most remarkable of these fiery globes were observed on March 31, 1686, in Italy and Germany; July 9, 1686, in Saxony, visible for seven minutes; March 19, 1 719, in England, in brightness almost equal to the sun; December 11, 1741, in England, visible about one P.M., during a bright sun; November 26, 1758, in England, splitting asunder with a fearful noise; July 10, 1771, in France and England, where the ball must have been over 1000 feet in diameter; August 18, 1783, in England, France, and the Netherlands, about fifteen miles high, and 2500 feet in diameter; March 8, 1798. in Switzerland; October 23, 1805; &c. With respect to the one seen on the Main, June 4 and 5, 1737, its least distance from the earth was estimated at thirty-four miles, and its velocity at somewhat more than that of the earth. There is no year in which fire balls are not observed in some place or other. Among those from which masses of iron fell are to be reckoned the meteors of May 26, 1751, near Agram; December 14, 1807, in North America, of 500 feet in diameter; June 15, 1821, in France, about three P.M.; &c.
The preceding remarks will suggest a connexion as existing between the fire balls and the meteoric stones, or aerolites, as we call those stones and mineral matters which fall after the splitting of the former. Aerolites do not, however, always fall from fire balls; sometimes they are cast from a small dark cloud, which suddenly forms in a clear sky, with a noise resembling that of single discharges of cannon. In much rarer instances the sky was clear, and there was no noise. Since the time of Chladni scientific men have been convinced of the quite frequent occurrence of falls of stones of greater or less dimensions from the atmosphere; the earlier accounts of this nature were generally considered as fabulous. On the 16th of June, 1794, a shower of stones occurred at Sienna; on the 13th of December, 1795, a stone fell in England weighing fifty-six pounds; on December 13th, 1798, several stones fell from a great fire ball; and on the 26th of April, 1803, a great shower of stones occurred near Aigle in France, of which was verified and investigated by Biot. In France ten such falls were observed in twenty-six years (1790 to 1815), from which it has been calculated that on an average 700 take place in the year, over the whole surface of the earth, or nearly two daily. In 1803 there fell at Aigle about 2000 fragments of stones, weighing from two drachms to seventeen and a half pounds each, over a surface of two and a half French miles in length, and one mile in breadth masses examined immediately after their fall were hot, some still glowing, and many exhibited traces of impression or indentation. The depth to which these stones bury themselves in the earth is very various; the greatest known is that of the mass weighing seventy-one pounds, which fell at Agram on the 26th of May, 1751; its diameter amounted to eighteen feet.
The shape of meteoric stones varies very much, in general it appears to be based on that of an inequilateral three or four-sided prism, or a distorted pyramid. The surfaces of the stones are rarely smooth, generally curved in such a manner that the convexity of one side corresponds to the concavity of the other; larger or smaller indentations are frequently seen on the outside. A characteristic of all meteoric stones is a peculiar thin, black rind, sometimes over a quarter of a line thick; they are occasionally of a pitchy lustre, and sometimes veined, frequently soft at first, discolored, or dusty.
The internal structure of different meteoric stones exhibits some general resemblances, and at the same time great differences. Some are very porous, absorbing water very readily; others are very compact. The specific gravity varies from 1.94 to 4.28, being at a mean about 3.5. Some masses contain not less than .96 of pure metallic iron, with a little nickel; of this character was the mass weighing 1400 pounds, found in Siberia in 1749, as also the Mexican specimen found at Zacatecas or Durango, weighing from 30,000 to 40,000 pounds, and other masses in all probability of meteoric origin. (The largest known aerolites, next to the Mexican just mentioned, are, one found in Bahia, and one near Otumpo in the province of Chaco, each from seven to seven and a half feet long, and weighing, the former 30,000 pounds, the latter 14,000 pounds.) Other aerolites again contain but two per cent, of iron, and some no metallic iron at all. We may therefore divide them into two classes: nickeliferous meteoric iron, and meteoric stones, properly so called. The metallic iron interspersed in almost all gives them a peculiar character; as for the rest, we find in meteoric masses the same chemical elements that occur in the earth’s crust, namely, eight metals: iron, nickel, cobalt, manganese, chromium, copper, arsenic, and tin; five earths: also potash, soda, sulphur, phosphorus, and carbon; in all eighteen, or about one third of the known elementary bodies.
With respect to the actual nature and origin of all these phenomena, the majority of investigators agree with Chladni that they are of cosmical, not atmospherical origin, being in all probability small masses, asteroids, moving with planetary velocity, and revolving in space about the sun in elliptic or parabolic orbits. Should they come within the sphere of the earth’s attraction, they are drawn off their course, becoming luminous on entering our atmosphere. The earth’s attraction need not necessarily destroy the motion of all these bodies coming within its sphere; the only effect may be an alteration in the orbitual motion of the body around the sun. We may assume the existence of several meteoric currents, composed of innumerable small worlds following each other in a closed ring; the different currents probably intersect the orbit of our earth like Biela’s comet, and the earth must, among others, pass through two of these cui rents in August and November. These asteroids are in all probability distributed very unequally in these closed rings, so that there may be only a few crowded groups; such a supposition may explain the rarity of the more conspicuous phenomena of this character. It is, to be sure, very enigmatical that the meteoric masses commence to shine and to become inflamed at heights which are considered as destitute of air. It is also a question, among many others which we cannot answer, whether the particles which compose the dense mass of a meteoric stone are originally distinct from each other in a gaseous condition, and first commence to be drawn together at the time they begin to shine; also, whether from the small shooting stars a compact mass may fall, or only a meteoric dust. A hypothesis, broached as early as 1660, suggests that meteoric stones may come from the moon, being ejected from volcanoes in active operation, a supposition readily refuted by the fact, as far as known, of the entire absence of active volcanoes on the moon. The older hypotheses of a telluric or atmospheric origin of meteoric masses are equally untenable.
Of the Electric Phenomena of the Atmosphere
Very soon after the discovery of electricity, attention was called to the remarkable similarity of its effects to those of lightning, particularly by Gilbert, Grey, Nollet, and Winkler. It was reserved for Benjamin Franklin to insist more fully on this identity, and to indicate the experiments by which this was to be proved, experiments performed nearly simultaneously by himself and others in France and England in 1752. Franklin made use of a paper kite with a hempen string, which he held in the hand. This apparent child’s play is for certain occasions, as when with a moderate wind it is wished to investigate the electricity of the higher strata, even yet the simplest and most applicable method. A great advantage is found in combining several kites into one system; nevertheless, in a highly excited atmosphere, this experiment becomes very dangerous. Other experimenters employed pointed iron rods, supported by insulating glass posts, and either standing freely in the air or else attached to some high building; this arrangement, however, is only calculated for intense electricity, as the glass posts soon lose their insulating property, owing to their becoming coated with dust or rain. An electrometer is used to measure the intensity of the fluid. Saussure armed the upper end of his electrometer with a wire two feet in length : it is still more advantageous to apply a flame to its point (or to attach a piece of burning sponge). A very useful piece of apparatus is a small Leyden jar of about ten or twelve square inches of inner coating, the conductor consisting of a metallic point projecting two inches above the jar, on which a metallic wire, of about three feet in length, with spiral turns, is attached, and often capable of being removed after charging the jar.
Traces of electricity are almost always found in the atmosphere, especially in clear weather: during a cloudless sky this electricity is always positive. The intensity of electricity in the same place is very variable, and subject to a regular oscillation. Feeble at sunrise, it increases until six and seven a.m. in summer, eight and nine in autumn and spring, and ten and eleven in winter. It then again decreases, reaching its minimum in summer about three, and in winter between four and six p.m.; at sunset it again commences to ascend, reaching a second maximum about one and a half or two hours after. This intensity likewise varies with the season, being greatest in the lower strata in January, and least in May, at the time when the air is driest. There is the most intimate connexion, as shown by Schiibler, between the daily and yearly periods of electricity and the variations of relative moisture. The intensity of positive electricity is also greater with the distance from the surface of the earth, as is shown by even slight differences in height. The intensity of electricity is remarkably great during the deposit of dew, and in fogs; also when after long continued bad weather it clears up suddenly, or when clouds have quickly formed and do not immediately separate. We sometimes find negative electricity in clouds and fogs, but only when rain has fallen from them. All water deposited from the air is more or less electric, the electricity being sometimes positive, sometimes negative; more frequently the latter. The intensity also is in proportion to the amount of condensation and the water falling in a given time. The direction of the wind is also of great influence: in north winds the rains are most frequently positively, and in south winds negatively electrified. In positive deposits the electricity is generally more intense than in negative, this electricity being usually far greater in amount in summer than in winter.
The most magnificent and at the same time most complex exhibition of electricity is furnished by thunder and lightning. Thunder clouds are generally small at first, but increase very rapidly, and soon cover the previously clear blue sky. Their color is in some places dark grey; under certain circumstances, however, exhibiting brilliant colors, particularly when, situated in a western sky, a declining sun tinges them with a yellow passing gradually into grey or blue. Generally a slow continuous falling of the barometer is observed previous to the formation of the cloud, accompanied in summer by an oppressive sultriness and a calm condition of the atmosphere. Should a thunder-cloud have formed in the vicinity of the zenith, a brisk wind rises at its approach, which blows in every direction from the cloud: in the cloud itself motions more or less lively are exhibited. and the electricity of the atmosphere quickly increases. The height of thunder clouds is sometimes very great, amounting occasionally to more than 20,000 feet. Should the charge of electricity in the cloud be sufficiently heavy, a flash of lightning will take place. Arago distinguished three kinds of lightning: 1. That consisting of a very fine and well defined luminous line, generally serpentine or zigzag. 2. That which illumines a great surface of the heavens at once; sometimes, however, only the outlines of those clouds from which it comes. 3. Lightning of greater duration than several seconds, of a well defined, generally spherical form; in this respect similar to the fire balls already described. The most frequent lightning is that of the second class. The color of lightning is sometimes white, sometimes bluish, violet, or red. A very deep red frequently characterizes lightning of the second class, whose light at any rate is generally less white and lively than that of the first. The duration of lightning of the first or second class is exceedingly short, not amounting even in the most brilliant and extended flashes to the thousandth part of a second. A division of a zigzag flash of lightning into two branches, very rarely into three, is some times observed. In many cases the discharge takes place between different strata of cloud, in others between a cloud and the surface of the earth: as a general rule, the flash comes from the cloud to the earth; sometimes, however, it passes from the earth to the cloud.
A noise of greater or less intensity, called thunder, generally accompanies the lightning. It differs greatly in its duration and character at different times, and appears to be much modified by the echo of terrestrial objects, and especially of mountains. When lightning strikes in our immediate vicinity, we hear first a sharp crack, and then a rattling sound in the distance. In other cases, particularly when the discharge takes place between the clouds themselves, there is heard a dull rolling or rumbling, sometimes lasting for several seconds, and occasionally swelling out and becoming stronger, and again fainter. In single cases the duration of the sound has been estimated at from half to three quarters of a minute. The longer duration of the thunder is explained by the time necessary for the effect of the lightning in the air to come to the ear, the path of the lightning often being of great length. There is generally a certain interval between the flash of lightning and the breaking forth of the thunder; this is because sound requires a longer time to traverse a given space than light, and in this way we may calculate the distance at which a visible discharge of lightning took place, by noting the time which elapses between the flash and the report. Allowing in round numbers 1000 feet for every second, the interval in some cases is less than half a second, in others forty to fifty, and in an instance cited by Arago seventy-two seconds.
Lightning without thunder often occurs in nearly clear as well as in cloudy weather. This heat lightning is seen in the horizon in low distant clouds, whose distance is too great to hear the accompanying thunder. The lightning seen at a little distance above the horizon shortly after sunset in a clear sky, appears not always to be accompanied by an explosion of thunder, but is at any rate an electrical phenomenon.
Lightning always follows the best conductors in its passage to the earth, and especially metals, in whatever way these may be covered by other bodies. As a general rule, little injury is produced, except on entering and leaving the mass, in which case the surrounding bodies are thrown about, torn up, pulverized, &c, the metal itself being partially melted. This is strikingly the case with wires which are not thick enough to allow of a ready passage to the fluid. Besides metals, lightning strikes men and animals, which are either killed or rendered senseless : these, next to metals, appear to be the best conductors, and after them, moist objects in general. Elevated bodies, particularly if they happen to be good conductors, are most apt to be struck, as towers, trees, steeples, &c. Sometimes what are called magic circles are seen in meadows, circles of three or four feet in diameter, where the grass has been singed. This appears to be owing to the lightning having fallen in considerable quantity. The second crop of grass from these circles is generally much fresher and greener than the rest of the meadow. When bad conductors are interposed in the path of the electric discharge, they are torn in pieces and scattered around, exercising not unfrequently an enormous power. Thus, in England, in the year 1809, a wall consisting of 7000 bricks, and weighing about twenty-five tons, was displaced from its position. In many cases part of the effect produced is to be ascribed to the sudden formation of highly elastic steam from the moisture of the conductor. We thus explain the fact that the green living tree, containing an abundant supply of sap, is more injured than a dead one, in which only a small portion of moisture exists. When combustible bodies are struck, they are generally set on fire; sometimes, however, only carbonized or shattered, in which latter case we sometimes speak of a cold stroke. The stroke is generally accompanied by a peculiar smell, due probably to the sudden formation of ozone.
Whether the lightning actually penetrates into the body of the earth on striking, or whether it merely becomes diffused over the surface, depends in every instance upon the peculiarities and conducting power of the surface, and upon the bodies subjacent. Such bodies are oftentimes considerably affected, becoming melted, glazed, or otherwise altered. When lightning strikes loose sand, it frequently marks its passage by the formation of long-tubes composed of the melted sand (known as lightning tubes or fulgurites). which are found in various regions of the earth. They generally present the appearance of a tube of unequal diameter in different parts, contracted interiorly, and then running out into a point. The outer surface is generally rough and sandy; the inside, however, well fused and smooth, and of a greenish color. The length amounts to from twenty to thirty-five feet, with lateral branches of from an inch to one foot; the diameter from three quarters to twenty French lines; and the thickness of the walls from one quarter to eleven lines. All these tubes that have been followed to any distance appear to lead to water. Even on the surface of hard rocks a glazing is sometimes noticed, probably produced by lightning.
One striking effect of lightning consists in its affecting those magnetic needles in whose vicinity it passes, sometimes reversing the poles, and even altogether destroying their magnetism. Under the same circumstances magnetism may be communicated in greater or less intensity to unmagnetized bars of iron or steel. These phenomena only confirm the belief in the electrical character of lightning.
A curious action of atmospheric electricity, to which attention has recently been called by Professor Joseph Henry, is the effect produced upon the magnetic telegraph. Not only are the wires often struck by direct flashes of lightning, and destroyed or injured, but an inductive influence is exerted by distant clouds, which sometimes causes an almost fearful play of the register. In many instances thunder storms have recorded their own approach on the fillet of paper long before there was any other evidence of the fact. And, indeed, in some cases the same effect has been produced without the agency of electrified clouds, but simply by the different electrical conditions of the strata of air through which the wires pass.
Besides the direct stroke of a lightning discharge, we also have the returning stroke, by which all the terrible effects of lightning may be produced at a considerable distance from the place where the preceding direct discharge had taken place. This phenomenon is explicable on the theory of electrical induction. The simplest case is that in which a large and heavily charged cloud electrified the earth by induction; on the discharge of the former the latter again is restored to equilibrium by the diffusion of the electricity which had been heaped up, this diffusion, when taking place through bad conductors, producing all the mechanical effects of ordinary lightning. The returning stroke is on the whole less dangerous than the other, and no credible instance has been adduced of its inflaming bodies.
Of all parts of the earth, thunders and lightnings are most abundant in the torrid zone during the rainy season (especially at the beginning and end). They here succeed each other almost daily at the times of maximum heat, while the lightning appears to be sharper as well as more luminous than in more temperate regions. With respect to the annual distribution of thunder and lightning in higher latitudes, the comparisons of Kaemtz show that thunder storms occur on an average about nineteen or twenty times each year in France, Holland, and Germany. Of these most take place in summer, the relative proportion in Germany being sixty-six per cent, in summer, twenty-four and a half in spring, eight in autumn, and one and a half in winter. In Russia and other interior portions of the old world, winter thunder storms are entirely wanting; there are seventy-nine per cent, in summer, sixteen in spring, and five in autumn; the total number appears to be less than in Germany and France. In Scandinavia the number of thunder storms is still less, continually decreasing towards the north. In the highest northern regions whole years elapse without thunder being once heard. On the west coast of Norway, particularly in the bishopric of Bergen, where about six thunder storms occur in the year, the winter storms predominate, these on the other hand being entirely wanting in Sweden. In Iceland, also, and on the western coast of North America, the winter thunders are most numerous; the Faroes, the Hebrides, and the Shetland Islands, not being entirely free from them. In Germany summer and winter thunders are distinguished by the greater poverty of lightning in the latter, and in frequently accompanying regular storms, while the former almost always arise in calm weather. Thunder and lightning are especially prevalent in mountainous regions, where a peculiar phenomenon is presented in this respect, namely, that a mountain crest or peak often forms a dividing line, beyond which such weather does not pass. Wooded mountains seem better adapted than bald for this purpose. It is especially the case, where a valley divides into several branches, a steep mountain standing in the forks of division, that storms coming up the valley often tarry about the mountain, and subsequently divide.
On Lightning Rods
A lightning rod is a contrivance invented by Franklin for conducting a stroke of lightning over or along a building or object of any kind, without any of the terrible effects of this powerful agent being exhibited. As lightning always follows the best conductor, it is very reasonable to suppose that a continuous rod of metal, of sufficient thickness, would carry it over a certain space without producing any injury to the poorer conductors in the immediate vicinity. Another suggestion of Franklin, that the electricity of a cloud may be silently drawn off by a pointed conductor, and a stroke thus averted, met with a great many opponents. It has been especially objected that the points of lightning rods, from their small extent and surface, must be incapable of carrying off a powerful discharge without injury; in fact, it has not rarely been found that they have actually been melted, and even the replacing the points by a better conducting metal, as copper or brass, cannot always avert this result.
The various arrangements employed for lightning rods may be divided into three classes: 1. Conductors of metal strips; 2. Conductors of metallic wire; and 3. Conductors of iron rods, Furthermore, we distinguish in each conductor three principal portions: the highest part where the lightning discharge is received; the middle part; and the inferior portion, or the end. Reimarus advises to carry a continuous metallic strip along the comb and eaves of the roof, across the gable ends, up the chimneys, and down the corners of the house. For this purpose, sheet lead of the proper thickness is very well adapted. In this case a special pointed conductor is not necessary. Reimarus recommends its use only in the case of thatched roofs. Should rods be deemed necessary, they must, according to him, be erected at the most exposed places, especially the chimneys and gables, to a height of about four feet, without points, and of at least three quarters of an inch in thickness. The communication between the strips of lead just mentioned and the ground, may best be established by means of copper or lead strips, about three inches wide, nailed to wood. To prevent the oxydation of the metal, it should be covered with a good coat of oil paint. It is unnecessary to separate the conductor from the building by iron or wooden pins or clamps. It is not advisable to inclose the conductor in the masonry or other inner portion of the building. The lower end of the conductor should, if possible, dip into open, and, indeed, running water, to assist in the diffusion of the electricity. When the extremity of the conductor leads into a covered channel of water, the inflammable gas which sometimes is present may be set on fire by the lightning, thus producing an explosion. It is advisable to have the conductor end in several branches, to multiply the points of egress. The conductors of vessels are best constructed of thin brass chain, or still better, of copper, linked together in joints of about six or eight inches long.
Saussure recommended conductors of brass wire. They have been extensively employed in Bavaria, without presenting any very pre-eminent advantages. Conductors of iron rods are not only the longest known, but also the most generally used. The Academy of Sciences at Paris, together with most French philosophers, recommend them above all others.
Professor Henry has suggested a very simple and effectual method of protecting a house, without much expense. It consists in employing the spouting as part of the conductor, by having the projecting rod connected with the gutter above, and leading a thick wire or iron rod from the lower end of each spout to running water or other good conductor. As most houses have a system of gutters along all the eaves, connected with the ground by several spouts, it would seem that this is an excellent, safe, and economical plan.
According to Pouillet, every lightning rod must consist of two essential portions, a pointed metallic rod projecting in the air, and a good conductor connecting the rod with the ground. To be of the greatest efficacy, the rod must terminate in a very fine point. The connexion with the ground must be perfectly conducting, no interruption of continuity taking place between the point and the ground; and all parts of the apparatus must be of the proper dimensions. The great advantage of a point consists, according to Pouillet, in this: that when a thunder cloud passes over the rod and decomposes its combined electricity by induction, by repelling the like and attracting the like kind, the latter can stream out into the air from the point. In this way no accumulation of electricity in the rod can take place, and no danger will be experienced in approaching it, or even from coming into actual contact.
Pl. 27, figs. 14–19, represent a lightning rod as recommended by Gay Lussac. This rod is about twenty-seven or twenty-eight feet long, and consists of three pieces, an iron rod 25f feet long, a brass piece of two feet, and a platinum needle of from one to two inches in length, together forming a cone tapering gradually above (fig. 15). The brass rod is screwed into the iron rod and secured by pins. The platinum needle is soldered to the brass rod by silver solder, and the joint surrounded by a copper nut, as in fig. 16. The iron rod consists sometimes, for more easy transportation, of two or more pieces screwed into one another and fastened by pins. Fig. 14 represents three different modes of attaching the rod to a building. Under the rod, about two or three inches from the roof, a plate, bb′ (fig. 17), is screwed to carry off the water; one or two inches above this plate the rod must be cylindrical and well turned, in order that a hinged ring, ll′ (figs. 17 and 18), may be applied, to which the conducting rod can be attached. This latter is a quadrangular iron rod of seven to nine lines in thickness, screwed to the ring ll′, and carried over the roof, and along the house to the ground. Here it should terminate in several branches and windings, dipping into a constant current of water, or into a hole bored to a depth at which water exists, and filled up with powdered charcoal. Should there be no water in any way accessible, the rod should at least be carried through a channel filled with charcoaf to a damp place. Instead of the conducting rod we may use a rope of twisted copper wire (fig. 19). A well-constructed rod of the dimensions just given, will protect a space of about sixty feet radius; and generally, a projecting rod will protect an area whose radius is twice the height of the rod. Should the rod project from the roof of a house, we must estimate the amount of protection extended to the house by the elevation of the rod above the roof.
The straw-rope hail and lightning conductor of La Postolle, pronounced perfectly useless on its first announcement, by the French Academy, has been already mentioned under the subject of Hail.
The inefficiency of all the earlier protective means, as the burning of great fires, the firing of cannon, &c., is now universally recognised. The ringing of bells, customary during thunder storms in olden times, and still practised in the Tyrol, is not only useless but dangerous to the persons concerned, who thus complete the electric communication between the bell, through the rope, and the earth. It has been estimated that in the short space of thirty-three years, not less than 386 church steeples have been struck, and 121 persons engaged in ringing the bells killed.
It still remains to mention an occasional electric phenomenon known at the present day as St. Elmo’s Fire (and to the ancients as Helena, or Castor and Pollux). During the disturbed condition of atmospheric electricity in storms, and at other times, flames are observed on elevated objects, such as metal points of towers, mast heads, &c, which are sometimes heard to crackle along these objects, but without doing any injury. This is nothing more than electrical light streaming forth during a great accumulation of free fluid, and is especially observed during violent storms, as also in snow and hail squalls. The ancients, when they saw two of these lights (Castor and Pollux) on the tops of the masts, considered them as indicative of fair weather, while a single one (Helena) was supposed to portend a storm. This phenomenon is presented more frequently in winter than in summer, and is sometimes exhibited in the former by a luminosity of the snow flakes, in the latter by the same peculiarity in the descending rain or hail. When the amount of free electricity is very great, it is seen on low objects, stalks of grass for instance, as observed by Burchell in South Africa; also on lance heads, canes, finger tips, ears and manes of horses, &c. The balls of fire sometimes observed, as distinguished from the flames or stars, may be the exhibition of negative electricity, the latter being positive.
Of Terrestrial Magnetism
The influence exerted by terrestrial magnetism, at any locality on the earth, is determined by measuring the magnetic declination, inclination, and intensity, as its three exponents. For the general consideration of this subject we would refer our readers to what is said on page 141. To determine the declination and its variations, the method of Gauss, and the accompanying apparatus, the magnetometer, are almost universally used. In this instrument, instead of small magnetic needles, magnetic bars of from five to twenty-five pounds’ weight are employed. A bar of this character, eighteen to thirty-six inches long, three to six lines thick, and fifteen to twenty-four lines broad, is placed on a nut of brass, which is suspended from the ceiling of a room by a fine wire, or thread of untwisted silk, about seven feet in length. A plane mirror is fastened to one end of the bar, whose plane stands perpendicular to the magnetic axis of the bar : opposite to the mirror, but at a distance of about sixteen feet, a telescope is attached, whose optical axis inclined slightly downwards is directed immediately towards the centre of the mirror. The angle made by the optical axis of the telescope with the plane of the astronomical meridian must be determined with the greatest possible accuracy. A horizontal scale about four feet long, graduated to millimetres, is attached to the stand of the telescope, perpendicularly to the direction of the magnetic meridian, and at such a height that the image of a part of it is seen by reflection in the mirror. A thread stretched by a weight, and in contact with the scale, hangs before the middle of the objective indicating the middle or zero point of the scale, that is, the point which lies in one and the same plane with the optical axis of the telescope. As security against currents of air, the bar hangs in a box having a small aperture in its movable cover to allow the passage of the thread, and another hole in the side opposite to the telescope for the mirror towards which the former is directed. Should the magnetic axis of the bar fall in the same vertical plane with the optical axis of the telescope, the image of the zero point of the scale will fall accurately in the axis or the cross-hair of the telescope; if this be not the case, the image of some other point of the scale than the zero point will appear in the latter, and when the distance of the scale from the mirror is accurately determined, we can readily calculate from the observed parts of the scale, the corresponding angle made by the magnetic axis of the bar with the optical axis of the telescope, and from the latter the corresponding declination. Nerther the inclination nor the intensity can be ascertained with the same exactness. See page 144 for the determination of the intensity.
Magnetic Observatories have been erected in various parts of the earth since 1828, thanks to the ceaseless efforts of Humboldt, observatories in which uninterrupted hourly observations are made for twenty-four or thirty-six hours in succession, at certain epochs. One of the most complete and best arranged establishments of the kind is the one in Greenwich, adapted also for making and registering meteorological observations. Pl. 27, fig. 20, represents this in ground plan, while fig. 21 presents a general view of the building as seen from the north. On the north side, a mast is erected to a height of eighty feet, intended for electrical observations. On the right side of the drawing a ball is seen on the ground, which, with its lantern, is intended for induction observations, and may readily be drawn to the top of the mast. The small building to the left is intended for observations on the magnetic inclination. The box not far from the door of the main building contains various thermometers, and may be turned so as to keep constantly in the shade. The main building itself, built of wood exclusively, without iron, and fourteen feet high, forms a cross of four equal arms or wings, which are erected according to the magnetic meridian, and, in the clear, are twelve feet broad and ten feet high. The distance between the extremities of tw r o opposite wings within the walls amounts to forty feet. The northern wing is separated from the middle space by a wall, thus forming a kind of antechamber. The letters of the plan indicate as follows: a the declination magnet in the southern wing, b the horizontal magnet in the eastern wing, c the vertical magnet in the western wing, d, e, f, three telescopes for observing the three magnets, a, b, c, from a single point (chair), o; g is the scale of b, h that of the vertical magnet, i a clock keeping mean time, l an astronomical clock, m a clock in the antechamber, k a barometer, n a chimney, p an alarm door bell, q a shed or offset for the electrical apparatus, ran opening in the roof in the direction of the astronomical meridian.
The declination magnet (pl. 27, fig. 24) is a thick magnetic bar of hardened steel, two feet long, one inch and a half broad, and one quarter of an inch thick; h is a brass ring with two plane glasses, between which are cross-hairs of spider’s web; d is the lower part of the attachment apparatus; e is a silk thread to which the magnet is suspended. This thread rises eight feet nine inches high, passes over two pulleys, f and g, and is attached by a piece of leather to the small reel, h, which, by the aid of a catch, permits the magnet to be elevated or lowered; i is a copper hoop, serving to restrain the oscillations of the magnet. The whole apparatus is placed on a metal stand, which rests on a particularly firm foundation. On the cross-arms is a rectangular box, coated inside and out with gold paper, in which the magnet swings freely.
The horizontal magnet is represented from the south-west in fig. 23. Here a is the magnet, b the mirror attached, c the circle of rotation, d five pairs of small pulleys, e, e, two silk suspension threads passing from the uppermost pair of pulleys to two pulleys, f, placed seven feet nine inches higher; thence they proceed over the pulley g, and finally to a larger pulley not shown in the figure. A catch wheel is attached to this latter pulley, its winch being shown at h. The magnet is similar in dimensions to the one already described, with a similar stand, eleven feet five inches high, and is surrounded by a copper hoop, i. Like the preceding it swings in a double box, whose south side consists partly of plate glass. A scale is attached to the wall of the eastern wing, about eight feet five inches south of the magnet, and is sighted by a telescope directed to the mirror, b, of the magnet. The magnet is placed in a direction perpendicular to the magnetic meridian, being held there by the tension of the threads, e, e. It strives continually to place itself in the direction of the magnetic meridian, turning the two threads, sometimes with greater, sometimes with less force, so that in consequence of the reflection from the mirror b, different numbers on the scale are constantly appearing.
The vertical magnet is seen in fig. 22. Here a is the magnet, b the mirror with adjusting screw placed on it, c a knife edge on which the bar turns, d one of the two agate plates on which c rests, e, e, screws by which the centre of gravity of the magnet and its inclination may be altered, f the bronze support on which the magnet rests. The latter is of like dimensions with the preceding, and is placed on a similar stand.
Pl. 27, fig. 25, exhibits the electrical apparatus placed in the window of the antechamber. In this, a is the hook which effects the connexion of the conducting wire with the apparatus, b is a screen covering the opening in the window, and through which there passes a vertical rod sustaining the apparatus, cc is a double truncated glass cone, fastened to the upper glass frame on each side by beds of brass, d, d, are lamps for keeping this glass cone constantly dry, e is a nut inclosing the glass cone, and carrying the hollow copper cylinder, gg, by means of the vertical arm, f. From this cylinder there pass out eight lateral arms, in which conducting rods can be moved freely up and down, and fixed at any part by screws. At h there is seen a Bohnenberger gold leaf electrometer, i is a galvanometer for determining the electric currents in the atmosphere, k is an instrument for measuring the length of the electric spark, l is a second dry pile apparatus, similar to h, but less sensitive, mm straw electrometers for determining electric changes in the atmosphere.
Finally, fig. 26 represents the electrical light apparatus, and in fact exhibits the apex of the electrometer, a is a lantern at the upper end, whose lamp burns constantly, b is a copper tube on which the lantern slides, fastened to the glass cone, c; this latter is hollowed beneath and coated with copper, under which stands the lamp marked e, constantly burning to heat the copper, and thus to maintain the glass cone in a proper condition of dryness; d is a wooden apparatus receiving the lower part of the cone, f is a conducting wire leading to the electrical apparatus in the antechamber, g, g are iron wires, by means of which the whole apparatus may be moved up and down.
The different values of the observed magnetic declination, inclination, and intensity, in different parts of the earth, are represented on charts by three systems of lines, called after Humboldt, isogonic, isoclinic, and isodynamic. Isogonic lines connect those parts of the earth possessing equal declinations. Charts on which they are delineated are called declination maps; they can, however, be relied on as accurate for a short time only, as the magnetic declination of a place is constantly changing. Nevertheless there are places on the earth where the declination does not sensibly change for a considerable period of time; among these are Spitzbergen and the western part of the Antilles. Among all the isogonic lines the line of no deviation is most remarkable, the line connecting all places where the needle points due north, or where the magnetic and astronomical meridians coincide. This passed through London in 1657, and through Paris in 1669. It divides the earth into two portions, of which the one has an eastern and the other a western deviation of the needle. The latter portion embraces all Europe, excepting a small part of Russia, Africa, and nearly all of the Atlantic Ocean. In north-eastern Asia the isogonic lines form a closed system of oval outline, this same condition being represented more regularly and of greater extent in the South Sea, between 20° N. and 45° S. latitude. In the Asiatic oval the deviation increases from without to within, while in that of the South Sea the reverse occurs. The chart occupying the middle of pl. 28 represents the observed values of the declination in the years 1827–30, in isogonic lines after Adolphe Erman. The figures accompanying the lines indicate the degree of declination, the western being taken as positive, the eastern as negative.
Isoclinic lines connect those parts of the earth possessing the same magnetic inclination, and are represented on inclination charts. That one of these lines connecting those places where the needle is horizontal or has no inclination, is called the magnetic equator; to the north of this the north end of the needle will dip towards the earth, the reverse taking place to its south. In 1825 the one point of intersection of the magnetic and terrestrial equators was situated near the Island of St. Thomas, in the western coast of Africa, and distant 188
Isodynamic lines connect those places which possess the same intensity of magnetism. Fig. 2 exhibits these lines for two hemispheres, likewise after Admiral Duperrey. In general the magnetic intensity increases from the terrestrial equator to either pole, but the isodynamic lines run parallel neither with the magnetic nor the terrestrial equator. An arbitrary unit has been made of the intensity observed at the magnetic equator in Peru by Humboldt, although this is by no means the minimum of observed intensity: this real minimum amounts to seven tenths of that assumed as the standard, and occurs on the coast of Brazil. By connecting those places in each meridian in which the intensity attains its minimum, we shall have a line, called by Duperrey the magnetic equator, which, however, by no means appears to coincide with the aclinic line, or line of no inclination. The maximum of known intensity amounts to a little over two, and occurs near the south magnetic pole; the observed maximum is thus about twice the minimum. Near the magnetic north pole in Melville Island it only amounts to 1.6. (See pl. 28, figs. 1a and 1b, where the term “magnetischer (magnetic) equator,” is to be taken in the meaning of Admiral Duperrey.)
The oscillations of the magnetic needles depend much on the course of the sun, so that at one and the same place the time of day may be ascertained by the position of the needle. The hourly variations of declination increase in extent with the magnetic latitude or the distance from the magnetic equator; thus, in Middle Europe they amount to thirteen or fourteen minutes, and near the equator to but three or four minutes. In the whole northern hemisphere the north end of the needle appears on an average to move westwardly from 8
The aurora exerts a greatly disturbing influence on the magnetic needle, for which reason this remarkable phenomenon is now almost universally considered as magnetic in its character, although no satisfactory explanation of it has yet been suggested. In high latitudes it is far more frequent than in lower, occurring almost every night during certain seasons in the far north.
The aurora, mostly observed in winter, generally begins with a bright glow, at first white and then yellowish, in the northern part of the heavens; its shape is that of an arch inclosing a dark nebulous cloud, previously formed in the otherwise clear heavens, and appearing as a segment of a circle from eight to ten degrees in height, which does not obscure the stars. In the far north this segment appears brighter than with us, or is entirely wanting. The highest point of the arc generally deviates from five to eighteen degrees from the magnetic meridian, towards that side to which the magnetic declination of the place is directed. Sometimes there arise two or three bright arches. From one of these, generally the uppermost, there subsequently ascend streaks of light and groups of rays of different colors, alternately appearing and vanishing, and changing their place with greater or less rapidity, so that the entire mass of light appears to be in incessant motion, the whole heavens being sometimes filled with a flickering, tremulous light. The colors, in particular instances, pass from violet and bluish white through all shades into green and purplish red; black streaks, even, resembling a thick smoke, occasionally occur. These streaks of light sometimes ascend from the arch alone, sometimes simultaneously from many opposite points of the horizon. The rays at times converge towards that point of the heavens corresponding to the direction of the dipping needle, thus forming the so-called crown of the northern light (pl. 26, fig. 13), resembling the lantern of a dome, and only rarely coming to perfection, but always constituting the culminating point of the whole phenomenon. This soon after begins gradually to decrease, flames up a few times more, and then vanishes, leaving either a whitish gleam in the north, which lasts some time longer, or a light white cloud.
This phenomenon, mentioned by Aristotle and Pliny, was first called aurora borealis by Gassendi. in consequence of the one observed by him on the 12th of September, 1621. The fact that the aurora appears more frequently at certain times than at others, is well ascertained, without our being able to determine a regular periodical alternation. According to Mairan, the following is the rate of their occurrence in earlier times: 26 between 583 and 1354 A.D.; from 1446 to 1560, 34; from 1561 to 1592, 69; from 1593 to 1633, 70; from 1634 to 1684, 34; from 1685 to 1721, 219; from 1722 to 1745, 961; from 1746 to 1751, 28. From 1716 to about 1790 they were so frequent, that the Dutch philosopher, Muschenbroek, observed no less than 720 at Leyden and Utrecht; in Leyden 750 were observed in twenty-nine years, while in 1730, 116 in all were seen in different parts of the earth. After 1790 they became rarer, and it is only since about 1825 that they have been observed more frequently. The auroras of modern times, most conspicuous for their beauty, their wide distribution, and long duration, were those of the 7th of January, 1831, and 24th and 25th of October, 1847 (seen even in the southern latitudes of Italy and Spain). Mairan gives the following as periods during which no northern lights appear to have been observed: from 1465 to 1520; from 1581 to 1600; and from 1721 to 1686. According to Bertholon, there were forty-nine years in the seventeenth century without any aurora. Hansteen indicates twenty-four aurora periods since 502 A.D., of which the ninth between 541 and 603, the twelfth between 823 and 887, the twenty-second between 1517 and 1588, and the twenty-fourth between 1707 and 1788, were eminently distinguished by the brilliance and frequency of the phenomenon. According to this author they are most numerous about the time of the equinoxes. Very few instances are on record in which they appeared by day, as on September 9th, 1827, in England.
The northern lights are sometimes visible in the torrid zone, and even in the southern hemisphere, just as the southern lights or aurora australis have been seen in the northern. In Europe, however, the northern lights do not appear often to descend below 37° of latitude. They are rarer in Switzerland, South France, and South Germany, than in Holland and North Germany. They are more numerous in Great Britain and Ireland, increasing to the sixty-fifth or sixty-sixth degree of latitude. Diminishing again in number towards the pole, their chief region appears to be in Europe, between 60° and 66° N. L. They are far more frequent in North America, and are visible further south than in Europe and Asia. The line of daily appearance of the aurora commences, according to Horner, at about 60° N. L., and 70° longitude west of Ferro, and runs thence north-easterly through Baffin’s Bay, the point of Greenland, Iceland, and the northern part of Spitzbergen, attains its highest point at 60° east longitude, and then returns through the Siberian Arctic Ocean, and above Behring’s Straits, to its starting point. The estimates of the height of the aurora are mostly very uncertain, varying between several miles and 3000 or 4000 feet. The distance from the earth is in all probability very different at different times, the phenomenon occurring not merely within the limits of the atmosphere, but even in the region of clouds. Some of the more recent observers even believe that the streamers of the northern light can be moved by winds and other aerial currents.
The connexion between the aurora and magnetism is not at all doubtful, considering the remarkable variations produced by the former in the magnetic declination, inclination, and intensity, especially since Faraday has discovered that light may be produced by magnetic forces. Even the morning previous to an aurora the irregular action of the needle indicates a disturbance in the equilibrium of terrestrial magnetism, and the needle is not seldom affected by the occurrence of this phenomenon in places where nothing of it is visible. It is only in the far north, beyond the actual zone of auroras, and near the magnetic pole, that there is no longer any influence of the northern light on the needle. These polar lights may then be considered as magnetic storms, during which the disturbed magnetic equilibrium is again restored.
In ancient times, and even up to the middle of the last century, the aurora was explained by supposing that terrestrial exhalations were collected in the higher regions of the atmosphere, and there inflamed. Many philosophers considered it to be an optical phenomenon, produced by the reflection of solar light from the polar snow and ice, against the concave surface of the higher atmospheric strata, whence the rays were a second time reflected to the observer. According to De Mairan, it is produced by the coming of the earth at stated periods into the atmosphere of the sun, supposed to extend to the earth’s orbit, an entirely false supposition, as shown by what is said of the dimensions of this atmosphere on page 237. According to Euler, it consists of solar rays, which, by their violent impact against the finer particles of the atmosphere, carry these to a height of more than 4000 miles, the height at which he believed them to exist. According to Kirwan. with whom Volta and Parrot agree, the northern light is produced by hydrogen gas generated by decomposition on the earth, volcanoes, and other causes, especially near the equator, and carried by aerial currents towards the polar regions, there to become inflamed. Before this, however, Halley considered it to be produced by the influence of magnetic currents from the two poles, and his hypothesis, in a somewhat modified form, is at present maintained by the most eminent investigators.